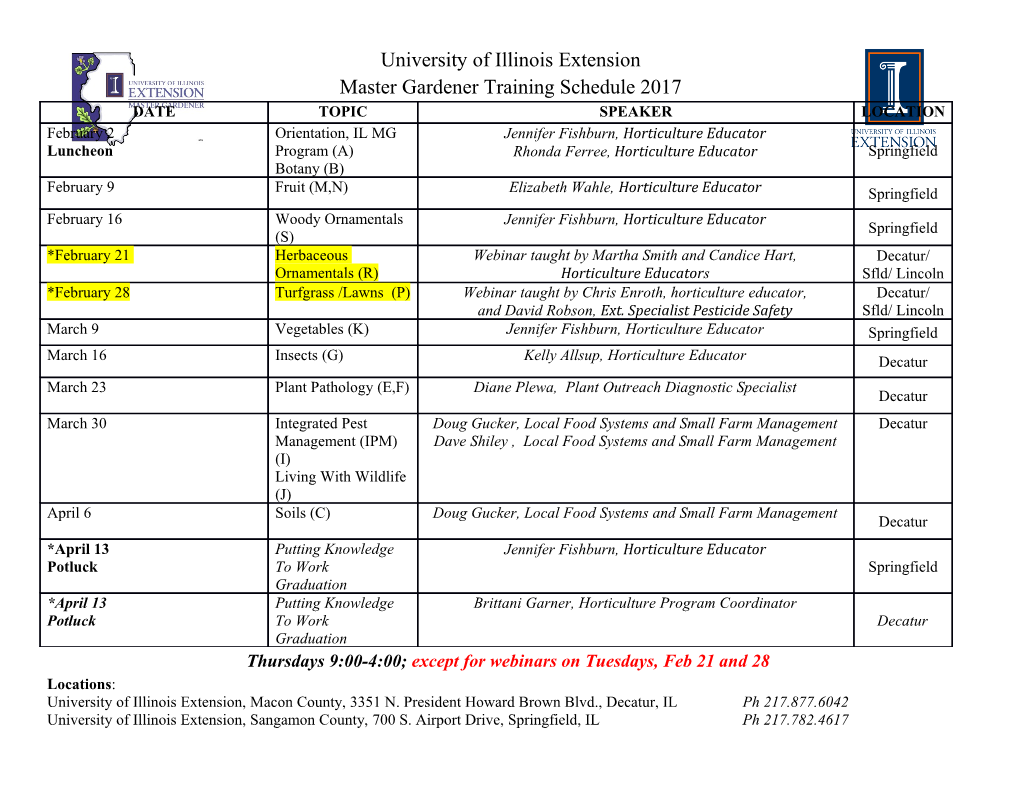
1 Combining shock barometry with numerical modeling: insights into 2 complex crater formation – The example of the Siljan impact structure 3 (Sweden) 4 5 S. Holm-Alwmark1*, A. S. P. Rae2, L. Ferrière3, C. Alwmark1, and G. S. Collins2 6 7 1Department of Geology, Lund University, Sölvegatan 12, 223 62 Lund, Sweden. 8 2Department of Earth Science and Engineering, Imperial College London, SW7 2BP, UK. 9 3Natural History Museum, Burgring 7, A-1010 Vienna, Austria. 10 11 * Corresponding author. E-mail: [email protected] 12 13 14 Abstract 15 Siljan, central Sweden, is the largest known impact structure in Europe. It was formed at 16 about 380 Ma, in the late Devonian period. The structure has been heavily eroded to a level 17 originally located underneath the crater floor, and to date, important questions about the 18 original size and morphology of Siljan remain unanswered. Here we present the results of a 19 shock barometry study of quartz-bearing surface and drill core samples combined with 20 numerical modeling using iSALE. The investigated 13 bedrock granitoid samples show that 21 the recorded shock pressure decreases with increasing depth from 15-20 GPa near the 22 (present) surface, to 10-15 GPa at 600 meters depth. A best-fit model that is consistent with 23 observational constraints relating to the present size of the structure, the location of the down- 24 faulted sediments, and the observed surface and vertical shock barometry profiles, is 25 presented. 26 The best-fit model results in a final crater (rim-to-rim) diameter of ~65 km. According to 27 our simulations, the original Siljan impact structure would have been a peak-ring crater. 28 Siljan was formed in a mixed target of Paleozoic sedimentary rocks overlaying crystalline 29 basement. Our modeling suggests that, at the time of impact, the sedimentary sequence was 30 approximately 3 km thick. Since then, there has been around 4 km of erosion of the structure. 31 32 Introduction 33 Impact cratering was once considered a minor geologic process without significance for the 34 evolution of Earth. Now, after more than 50 years of space exploration, we know that almost 35 all solid bodies in the Solar System display evidence of impact. The process is now known to 36 play a key role in the evolution of the Solar System, dominant in the formation of 37 planetesimals, and later planets, and it also most likely played an important role in shaping the 38 early crust of both Earth and other planets. Later in Earth’s history, impact cratering has 39 caused major environmental crises, even leading to at least one major mass extinction event, 40 at the Cretaceous-Paleogene boundary (Alvarez et al. 1980; Schulte et al. 2010). 41 In order to understand the major processes involved in impact cratering, a combination of 42 observations of impact structures, laboratory experiments, and numerical modeling has 43 proved to be successful, answering questions on the development of the transient cavity into 44 the final crater, the displacement of target rocks and formation of impactites, the ejecta 45 process and the induced environmental effects (e.g., Melosh 1989; Pierazzo et al. 1998; 46 Melosh and Ivanov 1999; Collins et al. 2004; see also Osinski and Pierazzo 2013, and 47 references therein). However, some major questions regarding the crater-forming process 48 remain unanswered, e.g., how the energy of the impact is distributed in the target rocks, and 49 the details on how geologic materials behave under the extreme conditions prevailing during 50 crater forming processes. Also, more specific questions relating to the formation of complex 51 impact structures, with the genesis of central uplifts, remain to be answered. 52 Understanding the formation of impact structures, especially large ones, is complicated 53 due to the fact that the process has never been directly observed. Laboratory experiments 54 cannot replicate the exact conditions prevailing due to physical limitations, and in addition, 55 impact structures on Earth are rapidly obscured by tectonic processes, erosion, and 56 sedimentation, complicating their study. For these reasons, numerical modeling of the 57 formation of impact craters has become an increasingly important and successful tool to 58 understand the mechanisms behind crater formation, and in describing individual impact 59 structures (e.g., Collins et al. 2002; Collins & Wünnemann 2005; Wünnemann & Ivanov 60 2003; Goldin et al. 2006; Ferrière et al. 2008; Morgan et al. 2016; Rae et al. 2017). However, 61 the success of the numerical simulations relies on being able to ground-truth them against 62 actual observations made from impact structures and/or samples subjected to shock 63 metamorphism. One way to do this is to compare the distribution of modeled shock pressures 64 in an impact event with determined shock pressures in physical samples from the modeled 65 impact structure (e.g., Rae et al. 2017). Determining peak shock pressures that rocks have 66 experienced can be achieved by characterizing the abundances and properties of shock 67 metamorphic features in rock-forming minerals of the target rocks. Previous detailed shock 68 barometry studies include the Charlevoix, Manicouagan, Slate Islands, Puchezh-Katunki, 69 Bosumtwi, Siljan, and West Clearwater Lake impact structures (see e.g., Robertson 1975; 70 Grieve and Robertson 1976; Dressler 1990; Fel’dman et al. 1996; Ferrière et al. 2008; Holm 71 et al. 2011; Rae et al. 2017). 72 In this paper, we present the results of a detailed study of samples from two drill cores 73 combined with numerical modeling to gain information on the characteristics of the large and 74 deeply eroded Siljan impact structure (Sweden). Our goals were to better constrain the 75 original size and morphology of the impact crater, to estimate the pre-impact sedimentary 76 sequence overlying the crystalline bedrock in the area, and to evaluate the amount of post- 77 impact erosion. We constrain our numerical simulations by comparison with the presently 78 observed structure, as well as the results of shock barometric analysis across the present 79 erosional level of Siljan that was presented by Holm et al. (2011), and also drill cores from the 80 structure (this study). This comparison provides robust insights into complex crater formation. 81 82 83 Geological Setting 84 The Siljan impact structure, located in the Dalarna region of central Sweden, consists of a 85 central area with a diameter of 28-30 km surrounded by a partly lake-filled annular depression 86 (Fig.1). The structure lacks original crater morphology due to extensive erosion exposing 87 rocks that were originally located below the crater floor (Grieve 1988). The apparent annular 88 depression is thus also an effect of preferential erosion, and not an original crater feature 89 (Holm et al. 2011). The central area, and the area to the west of the structure, is dominated by 90 Dala granites of Järna and Siljan types, intruded as part of the Trans-Scandinavian Igneous 91 Belt (intrusion ages 1.85-1.65 Ga; Högdahl et al. 2004 and references therein), with rare 92 occurrences of mafic intrusive, extrusive, and sedimentary rocks. To the east of the structure, 93 granitic Svecofennian rocks of older ages dominate (2.1-1.87 Ga; Högdahl et al. 2004 and 94 references therein). The impact structure was formed in the end of the Devonian period, at 95 380.9 ± 4.6 Ma (Reimold et al. 2005; Jourdan et al. 2012). 96 Inside the 10 km wide annular depression, Paleozoic sedimentary rocks constituting a 97 megabreccia of m- to km-sized blocks (Collini 1988) have been preserved. The apparent 98 stratigraphic thickness of the sedimentary sequence varies around the structure, with 99 maximum values of ~600 m in the western part of the Siljan ring (Juhlin et al. 2012; Lehnert 100 et al. 2013). At the time of impact, the thickness of the overlying sedimentary rocks/sediments 101 is not known, a fact relating to the absence of exposed Paleozoic sedimentary rocks outside of 102 the Siljan ring, due to erosion. This sequence has been quoted as being 400-500 (or even 650) 103 m thick at the time of impact (Rondot 1975; Collini 1988; Vlierboom et al. 1986; Lindström 104 et al. 1991). However, a substantially thicker sedimentary sequence associated with a thick 105 Caledonian foreland basin, up to 4 km, has been suggested based on studies of thermal 106 indicators, such as δ18O/δ13C, conodont alteration indices, and fission track data (Tullborg et 107 al. 1995; Larson et al. 1999; Cederbom et al. 2000; Cederbom 2001). The interpretation of 108 Larson et al. (1999) and Cederbom et al. (2000) based on fission track data, of a several km 109 thick Caledonian foreland basin was challenged by Hendriks & Redfield (2005), who 110 suggested that there is no evidence, either from fission track, or from the geological data of 111 the region, for a substantial Caledonian foreland basin covering Fennoscandia, prompting a 112 so-far unresolved discussion (Larson et al. 2006; Hendriks & Redfield 2006). The large 113 discrepancy in the estimated thickness of the sedimentary unit in the Siljan area, at the time of 114 impact, is a result of uncertainty regarding the location of Siljan in relation to the foreland 115 basin and bulge of the Caledonides, along with the exact quantity of erosional products 116 transported to the Caledonide foreland basin being unknown. At the time of impact, this 117 sedimentary sequence was most likely composed of unconsolidated sediments overlying 118 consolidated sedimentary rocks. However, the relative thicknesses of each is not known and, 119 as we discuss later in the paper, we use both terms to describe this unit of sediment/rock.
Details
-
File Typepdf
-
Upload Time-
-
Content LanguagesEnglish
-
Upload UserAnonymous/Not logged-in
-
File Pages45 Page
-
File Size-