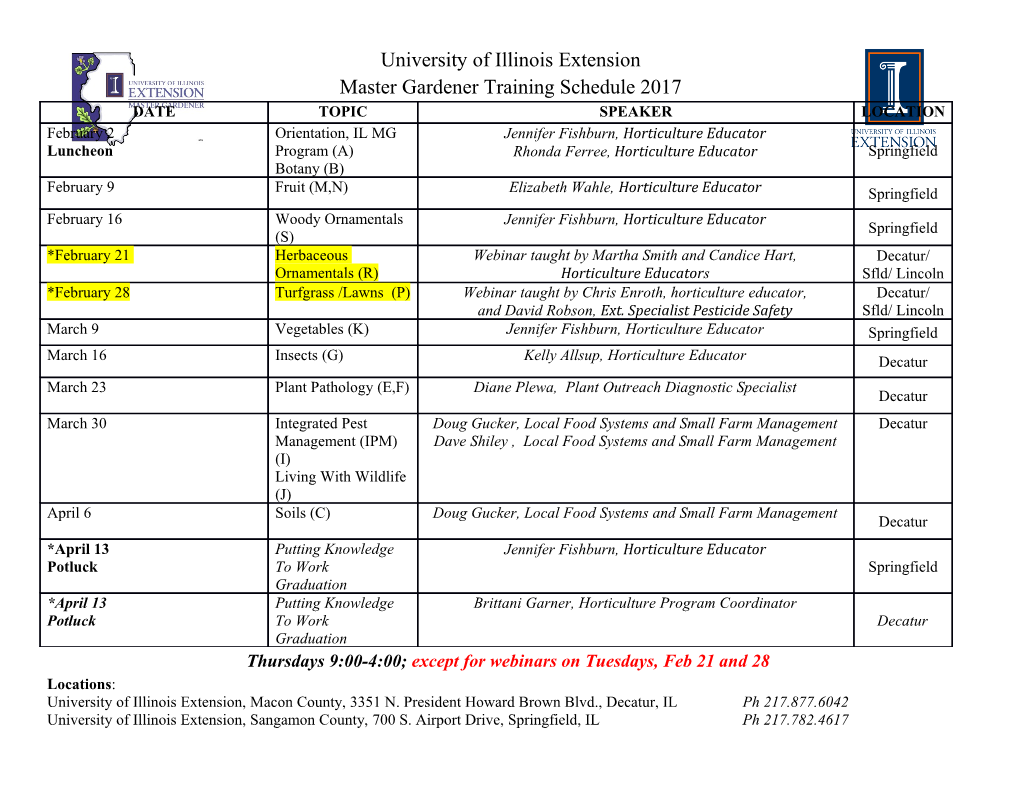
Meteoritics & Planetary Science 1–19 (2020) doi: 10.1111/maps.13541 High-resolution microstructural and compositional analyses of shock deformed apatite from the peak ring of the Chicxulub Impact Crater Morgan A. COX *1,2, Timmons M. ERICKSON 1,3, Martin SCHMIEDER 2, Roy CHRISTOFFERSEN3, Daniel K. ROSS3, Aaron J. CAVOSIE 1, Phil A. BLAND 1, David A. KRING 2, and IODP–ICDP Expedition 364 Scientists 1Space Science and Technology Centre (SSTC), School of Earth and Planetary Science, Curtin University, Perth, Western Australia 6102, Australia 2Lunar and Planetary Institute (LPI)—USRA, 3600 Bay Area Boulevard, Houston, Texas 77058, USA 3Jacobs-JETS, Astromaterials Research and Exploration Science Division, NASA Johnson Space Center, Houston, Texas 77058, USA *Correspondence. E-mail: [email protected] (Received 01 December 2019; revision accepted 09 June 2020) Abstract–The mineral apatite, Ca5(PO4)3(F,Cl,OH), is a ubiquitous accessory mineral, with its volatile content and isotopic compositions used to interpret the evolution of H2Oon planetary bodies. During hypervelocity impact, extreme pressures shock target rocks resulting in deformation of minerals; however, relatively few microstructural studies of apatite have been undertaken. Given its widespread distribution in the solar system, it is important to understand how apatite responds to progressive shock metamorphism. Here, we present detailed microstructural analyses of shock deformation in ~560 apatite grains throughout ~550 m of shocked granitoid rock from the peak ring of the Chicxulub impact structure, Mexico. A combination of high-resolution backscattered electron (BSE) imaging, electron backscatter diffraction mapping, transmission Kikuchi diffraction mapping, and transmission electron microscopy is used to characterize deformation within apatite grains. Systematic, crystallographically controlled deformation bands are present within apatite, consistent with tilt boundaries that contain the <c> (axis) and result from slip in <1010 > (direction) on f1120g (plane) during shock deformation. Deformation bands contain complex subgrain domains, isolated dislocations, and low-angle boundaries of ~1° to 2°. Planar fractures withinÈÉ apatite form conjugate sets that are oriented within either {2110g, {2110g,{1120g,or1120 . Complementary electron microprobe analyses (EPMA) of a subset of recrystallized and partially recrystallized apatite grains show that there is an apparent change in MgO content in shock-recrystallized apatite compositions. This study shows that the response of apatite to shock deformation can be highly variable, and that application of a combined microstructural and chemical analysis workflow can reveal complex deformation histories in apatite grains, some of which result in changes to crystal structure and composition, which are important for understanding the genesis of apatite in both terrestrial and extraterrestrial environments. INTRODUCTION high-pressure/-temperature polymorphs must be identified and documented (e.g., French 1998; French and Impact cratering has played a major role in shaping Koeberl 2010). Shock deformation microstructures and reworking planetary bodies within our solar system within minerals are, in many cases, a reliable indicator of (e.g., Baldwin 1963; Shoemaker 1983; Melosh 1989). In pressure conditions experienced by target rocks during an order to confirm an impact structure on Earth, the impact event. Shock-produced microstructures in presence of shatter cones, meteoritic components, shock common crustal minerals such as quartz, feldspar, and deformation microstructures within minerals, and/or zircon have been studied extensively (e.g., Stoffler¨ and 1 © The Meteoritical Society, 2020. 2 M. A. Cox et al. Langenhorst 1994; French 1998; Timms et al. 2017), clasts largely retain their pre-impact basement ages while the response of other accessory minerals such as (McGregor et al. 2018). Cernokˇ et al. (2019) correlated titanite, apatite, monazite, and xenotime has received less phosphate microstructures with different shock stages attention (e.g., Cavosie et al. 2016; Erickson et al. 2016; using coexisting feldspar and electron backscatter McGregor et al. 2018; Cernokˇ et al. 2019; Timms et al. diffraction (EBSD) mapping of apatite. They showed 2019; Kenny et al. 2020). Here, we present a detailed that as shock pressure increased, deformation in apatite study of shock deformation in apatite from granitoid and transitioned from a brittle deformation regime to the impact melt lithologies in the recently drilled peak ring of crystal–plastic regime with low-angle boundaries, the Chicxulub impact structure. followed by subgrain formation and the loss of crystallinity (Cernokˇ et al. 2019). Similarly, Kenny et al. Shock Deformation in Apatite (2020) showed that apatite grains from a clast-rich impact melt rock (with lithic and mineral clasts that Shock deformation microstructures in apatite experienced >35 GPa; Schmieder et al. 2008) show described previously include planar fractures (PFs) evidence for shock recrystallization and intragrain (Cavosie and Centeno 2014; Sløby et al. 2017; crystal–plastic deformation. McGregor et al. 2018; see discussion in Montalvo et al. 2019), recrystallization (Alwmark et al. 2017; McGregor Chicxulub Impact Structure et al. 2018, 2020; Cernokˇ et al. 2019; Kenny et al. 2020), microvesicles (Wittmann et al. 2013; McGregor The Chicxulub impact structure, located on the et al. 2018), crystal–plastic deformation (Cernokˇ et al. Yucatan´ Peninsula of Mexico, is ~180 km in diameter 2019; Kenny et al. 2020), and cataclastically deformed and exhibits a well-preserved peak ring (Fig. 1) (e.g., zones (Birski et al. 2019; Cernokˇ et al. 2019). PFs Hildebrand et al. 1991; Kring 1995, 2005; Morgan et al. consist of multiple sets of parallel, planar features that 1997, 2016; Gulick et al. 2008; Kring et al. 2004; Riller cut across the host apatite grain and are typically et al. 2018; Rae et al. 2019). The peak ring of the spaced 5–10 µm apart (Cavosie and Centeno 2014; Li structure is ~80 to 90 km in diameter and rises ~400 m and Hsu 2018; McGregor et al. 2018; Montalvo et al. above the structural crater floor (Fig. 1) (Gulick et al. 2019). PFs identified in apatite grains through 2013). The impact occurred ~66 Ma ago, produced backscattered electron imaging appear similar to those global ejecta deposits, and is causatively related to the identified in shock-deformed zircon and xenotime (e.g., K-T mass extinction event (e.g., Alvarez et al. 1980; Kamo et al. 1996; Cavosie et al. 2010, 2016; Erickson Kring and Boynton 1991; Swisher et al. 1992; Smit et al. 2013; Cavosie et al. 2016). PFs in apatite have 1999; Kring et al. 2017; Schulte et al. 2010; Renne et al. been interpreted to form in the { 1011} orientation 2013, 2018; Sprain et al. 2018). Based on the presence (Cavosie and Centeno 2014), which is different from of impact melt rock and shock metamorphic features natural cleavage directions, {0001} and {hki0}, of identified in early drill core materials (samples from the apatite (Deer et al. 2013). Dislocations and PFs in Yucatan-6´ borehole), Chicxulub was confirmed to have apatite have been produced experimentally within formed from a hypervelocity impact event (e.g., apatite shock-loaded to ~25 GPa using a plate-wave Hilderbrand et al. 1991; Kring and Boynton 1991; generator (Sclar and Morzenti 1972). In natural Sharpton et al. 1992, 1996). samples, PFs in apatite have been identified in rocks In 2016, the International Ocean Discovery that have experienced ~10 to 20 GPa at the Santa Fe Program (IODP) and International Continental impact structure (New Mexico, USA; Cavosie and Scientific Drilling Program (ICDP) drilled 829 m of core Centeno 2014; Montalvo et al. 2019), as well as in clasts from the peak ring of the Chicxulub crater during within impact breccia from the Nicholson Lake impact Expedition 364 (e.g., Morgan et al. 2016; Riller et al. structure (Canada; McGregor et al. 2018) that 2018). Borehole M0077A (21.45°N, 89.95°W) experienced ~10 GPa. encountered ~112 m of postimpact deposits, ~130 m of Shock-induced recrystallization has been reported reworked suevite and underlying impact melt rock, and within apatite from multiple terrestrial impact structures ~587 m of coarse-grained granitoid rocks of the such as Carswell (Canada; Alwmark et al. 2017), crystalline crater basement (predominantly ~340 Ma) Nicholson Lake (McGregor et al. 2018), and Paasselka¨ that also host pre-impact mafic and felsic volcanic (Finland; Kenny et al. 2020), as well as in phosphate dykes, veins and dykes of impact melt, and lithic minerals in lunar samples (Cernokˇ et al. 2019). Apatite (cataclastic) breccia dykes (Fig. 1) (e.g., Morgan et al. grains in contact with impact melt from the Nicholson 2016; Riller et al. 2018). Lake impact structure were shown to have partially Previously indexed orientations of planar reset U-Pb ages, whereas apatite grains with PFs within deformation features (PDFs) and PFs in quartz Shock deformation in apatite 3 Fig. 1. Location and geophysical images of the Chicxulub impact structure. A) Bouguer gravity anomaly of the crater modified from Rae et al. (2019). B) Seismic profile of the peak ring after Gulick et al. (2013). C) Schematic log of the peak-ring drill core showing sample locations, modified from Morgan et al. (2016). constrain shock pressures between ~15 and 18 GPa 1132.79; 1240.62; 1250.19; 1278.47; 1320.5; 1333.6; see throughout the basement portion of the core (e.g., data
Details
-
File Typepdf
-
Upload Time-
-
Content LanguagesEnglish
-
Upload UserAnonymous/Not logged-in
-
File Pages19 Page
-
File Size-