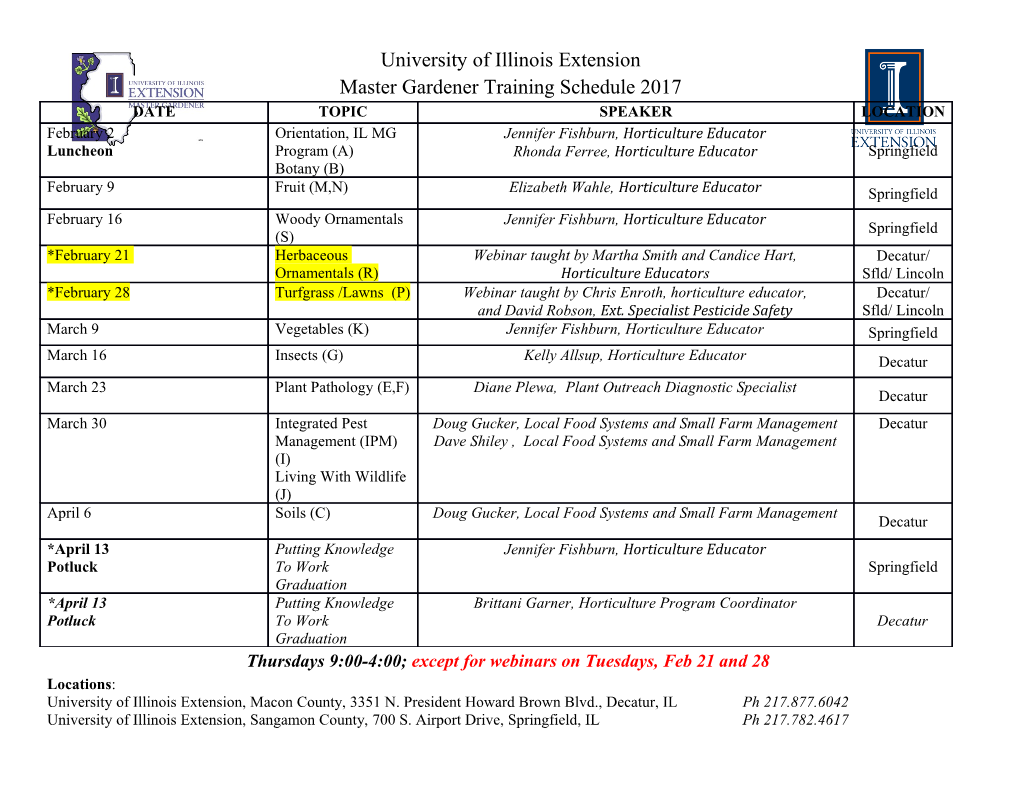
Revealing Electronic Signature of Lattice Oxygen Redox in Lithium Ruthenates and Implications for High-Energy Li-ion Battery Material Designs Yang Yua,*, Pinar Karayaylalib, Stanisław H. Nowakc, Livia Giordanob,d, Magali Gauthierd, †, Wesley Honga, Ronghui Koue, Qinghao LiF, John Vinsong, Thomas Krollc, Dimosthenis Sokarasc, *, Cheng-Jun Sune, Nenian Charlesd, Filippo Magliah, Roland Jungh, Yang Shao- Horna,b,d,* a Department of Materials Science and Engineering, MIT, Cambridge, MA 02139, USA b Department of Mechanical Engineering, MIT, Cambridge, MA 02139, USA c SLAC National Accelerator Laboratory, Menlo Park, CA, 94025, USA d Research Laboratory of Electronics, MIT, Cambridge, MA 02139, USA e Advanced Photon Source, Argonne National Laboratory, Argonne, IL 60439, USA f Advanced Light Source, Lawrence Berkeley National Laboratory, CA 94720, USA gNational Institute of Standards and Technology, Gaithersburg, Maryland 20899, USA h BMW Group, Petuelring 130, 80788 Munich, Germany †Current address: M.G.: LEEL, NIMBE, CEA, CNRS, Université Paris-Saclay, CEA Saclay 91191 Gif-sur-Yvette, France. Corresponding Authors * Yang Yu ([email protected]) * Dimosthenis Sokaras ([email protected]) * Yang Shao-Horn ([email protected]) 1 ABstract Anion redox in lithium transition metal oxides such as Li2RuO3 and Li2MnO3, has catalyzed intensive research efforts to find transition metal oxides with anion redox that may boost the energy density of lithium-ion batteries. The physical origin of observed anion redox remains debated, and more direct experimental evidence is needed. In this work, we have shown electronic signatures 2- n- of oxygen-oxygen coupling, direct evidence central to lattice oxygen redox (O /(O2) ), in charged 4+ 5+ Li2-xRuO3 after Ru oxidation (Ru /Ru ) upon first-electron removal with lithium de-intercalation. Experimental Ru L3-edge high-energy-resolution fluorescence detected X-ray absorption spectra (HERFD-XAS), supported by ab-initio simulations, revealed that the increased intensity in the high-energy shoulder upon lithium de-intercalation resulted from increased O-O coupling, inducing (O-O) !∗-like states with # overlap with Ru d-manifolds, in agreement with O K-edge XAS spectra. Experimental and simulated O K-edge X-ray emission spectra (XES) further supported this observation with the broadening of the oxygen non-bonding feature upon charging, ∗ also originated from (O-O) ! states. This lattice oxygen redox of Li2-xRuO3 was accompanied by a small amount of O2 evolution in the first charge from differential electrochemistry mass spectrometry (DEMS) but diminished in the subsequent cycles, in agreement with the more reduced states of Ru in later cycles from Ru L3-edge HERFD-XAS. These observations indicated that Ru redox contributed more to discharge capacities after the first cycle. This study has pinpointed the key spectral fingerprints related to lattice oxygen redox from a molecular level and constructed a transferrable framework to rationally interpret the spectroscopic features by combining advanced experiments and theoretical calculations to design materials for Li-ion batteries and electrocatalysis applications. 2 TOC 1. Introduction The redox process of positive electrode materials largely dictates the energy density and cycling stability of the Li-ion batteries. Conventional positive electrode materials including LiCoO2 and LiNixMnyCozO2, with layered structures, hinge on the reversible redox couples of 3d transition metals including Co3+/Co4+,1-2 Ni2+/Ni4+ (or Ni3+/Ni4+) 3-4 and Mn3+/Mn4+,5- 6 1- accompanied by lithium (de-)intercalation, delivering a capacity of around 140 mAh/g, 6 upon charging to 4.3 V with respect to Li metal (VLi). This redox process is rooted in the electronic structure of lithium early transition metal oxides. Due to their large electronegativity difference, oxygen 2p-states lie well below the transition metal d-states, hence, the Fermi level has a predominantly metallic character, and the transition metals mainly undertake the burden of the redox reaction.7-9 For most transition metals, this cationic redox scenario usually provides one electron per metal site and poses significant limitations on the energy density of positive electrode materials for mobile devices and automotive applications.9 However, as we move from early to late transition metals, or to higher valence metal states via lithium de-intercalation, we increase the electronegativity of the transition metal species.10 This results in a larger degree of energetic overlap between 3 the metal d-states and the oxygen p-states, and therefore a more covalent interaction between the transition metal and oxygen.8-9, 11 In this scenario a direct extraction/replenishment of the electron density from the oxygen density of states can occur.7-8, 12 This scenario is usually referred to as anionic redox, in contrast with conventional cationic redox. The notion of anion redox arose for the first time in LiCoO2 13 in the 1990s, where oxygen release was observed upon charging up to 4.4 VLi. Ceder et al.12 explained this finding using density functional theory (DFT) calculations in which both metal and oxygen contribute to the redox processes at high potentials.12 Within the 3d metals family, going from early transition metals to late transition metals, this oxygen charge compensation mechanism is augmented due to a more covalent metal-oxygen framework12. 8, 11, 14-22 Li-rich layered oxides, Li2MO3 (M = Ru, Ir, Mn), have been studied extensively and offer much higher energy density than LiMO2 by providing more lithium for (de-)intercalation. These oxides can be written generally as Li(Li1/3M2/3)O2, with 1/3 of the transition metal sites within the transition metal layer occupied by Li. This Li excess allows a higher transition metal valence (4+) , compared to LiMO2 (3+), and therefore leads to more overlap between transition metal and oxygen states, resulting in a highly covalent M- O bonding.7, 10, 12 These reported Li-rich metal oxides can be divided into two classes. The 17-18, 21, 23-37 first class is represented by oxides derived from Li2MnO3, which exhibits a relatively poor reversibility attributed to oxygen redox (oxidation) into molecular oxygen, leading to oxygen loss. Although Li2MnO3 can yield a first cycle discharge capacity higher 25, 29-32, 34-35 than 200 mAh/g, upon charging to 4.8 VLi, it suffers from a poor cycling 23, 29-31 17,32 performance. Considerable oxygen loss occurs upon charging Li2MnO3 , where 4 30 0.125 oxygens can be released per unit of extracted Li . The Li2MnO3 derived compounds such as Li(LixMnyNizCo1-x-y-z)O2 (Li-rich Mn-rich NMC) introduce additional redox couples (Ni2+/Ni4+ and Co3+/Co4+) that may boost both cationic and lattice oxygen redox, reaching a reversible capacity of around 300 mAh/g.36-38 Although their cycling stability is much improved compared to pure Li2MnO3 electrodes, they still suffer from a significant voltage decay over subsequent cycles37, and the oxygen redox contribution to the redox process is diminished upon extended cycling.37 The second group of Li-rich metal oxides 11, 39-41 42-43 is derived from noble metals including Li2RuO3 and Li2IrO3, which show reversible capacities with a two-electron transfer process and a high cycle life. For example, 15-16, 39-40 Tarascon and co-workers have shown that Li2Ru1-xMnxO3 and Li2Ru1-xSnxO3 deliver first discharge capacities as high as 250 mAh/g,16, 39-40 and capacity retention of higher than 80 % after 100 cycles.16, 39 It has been proposed that the capacity corresponding to the second electron transfer results from reversible lattice oxygen oxidation into peroxo- and superoxo- like species in the bulk.11, 16, 39-40 The mechanism giving rise to the reversible capacities associated with the lattice oxygen redox is not well understood. Unambiguous experimental evidence or an electronic structure signature for lattice oxygen redox for these Mn-, Ir- and Ru- based oxides is missing. There are primarily two schools of thought on the physical origin of lattice oxygen redox in Li-rich oxides. The first class of proposed mechanisms argues that anion redox n- 11, 39-41, 43 2- induces the formation of (O2) peroxo-like species, and the redox couple O / n- (O2) can proceed reversibly upon cycling. A certain M-O bond covalency is required for reversible oxidation of the anion as proposed by Doublet, Tarascon and coworkers.11, 41 n- Tarascon et al. have proposed the existence of peroxo-like O2 species for Li2-xRuO3 at 4.6 5 16, 39 VLi during the first cycle via X-ray photoelectron spectroscopy (XPS) studies. The formation of such peroxo-like species is accompanied by Ru reduction which is referred to as a reductive coupling mechanism.11, 40 The Ru6+ species are stabilized by modifying the coordination sphere of Ru through oxygen-oxygen bond formation and the transfer of extra 5+ 2- electrons to the transition metal for reduction, eventually forming Ru -(O2) peroxo- 4+ - species or Ru -(O2) superoxo-species. However, we should note that the XPS measurement has a penetration depth of only 5 nm to 10 nm44. Therefore, the evidence provided by XPS is from the surface and subsurface of the materials which is likely not representative of the bulk. Moreover, the XPS Fingerprint is not clear and cannot be unequivocally assigned to peroxo-like species, but might also come from surface electrolyte decomposition products. Hence, there is no unambiguous experimental evidence from an electronic structure perspective to support this proposed redox process. The formation of peroxo-like species is further demonstrated and visualized by McCalla et al.43 using high-resolution transmission electron microscopy and neutron diffraction in 43 delithiated Li2-xIrO3, where an O-O bond distance of around 2.4 Å has been shown. In addition, Saubanère et al.11 have reported from crystal orbital overlap population (COOP) analysis that the covalent bonding between Ru-O stabilizes the peroxo-like species in Li2- xRuO3 upon oxidation.
Details
-
File Typepdf
-
Upload Time-
-
Content LanguagesEnglish
-
Upload UserAnonymous/Not logged-in
-
File Pages56 Page
-
File Size-