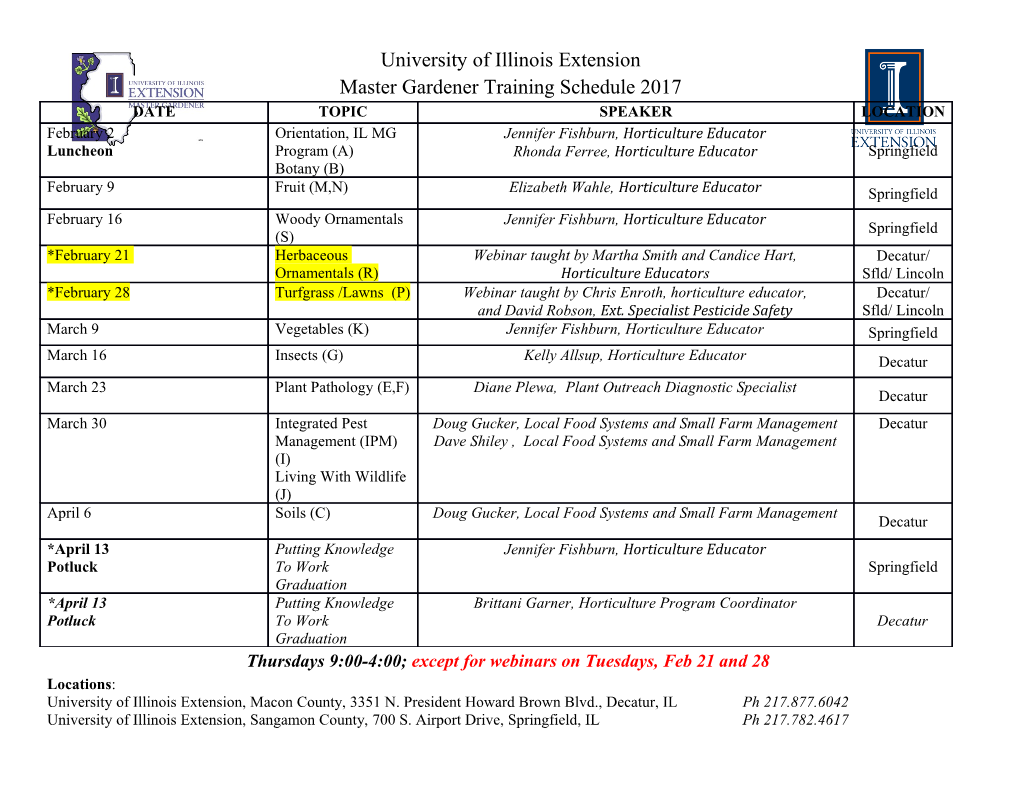
Strength of chrysotile-serpentinite gouge under hydrothermal conditions: Can it explain a weak San Andreas fault? D. E. Moore D. A. Lockner U.S. Geological Survey, Menlo Park, California 94025 R. Summers Ma Shengli Institute of Geology, State Seismological Bureau, Beijing, China J. D. Byerlee U.S. Geological Survey, Menlo Park, California 94025 ABSTRACT more, it has been suggested that at higher temperatures (Reinen et Chrysotile-bearing serpentinite is a constituent of the San An- al., 1993) and/or lower strain rates (Reinen and Tullis, 1995) the dreas fault zone in central and northern California. At room tem- frictional strength of chrysotile may be reduced to Յ 0.1. As a test perature, chrysotile gouge has a very low coefficient of friction ( of this hypothesis, we report here on the strength of a pure chrysotile Ϸ 0.2), raising the possibility that under hydrothermal conditions gouge at elevated temperatures and a wide range of velocities, in- might be reduced sufficiently (to <0.1) to explain the apparent tended to more closely represent conditions at depth in the fault. weakness of the fault. To test this hypothesis, we measured the Our results suggest that chrysotile alone is not a likely explanation frictional strength of a pure chrysotile gouge at temperatures to for a weak San Andreas fault. ؇C and axial-shortening velocities as low as 0.001 m/s. As 290 temperature increases to Ϸ100 ؇C, the strength of the chrysotile EXPERIMENTAL PROCEDURES gouge decreases slightly at low velocities, but at temperatures The chrysotile gouge used in this study was prepared from a ؇C, it is substantially stronger and essentially independent of soft, layered, light to medium grayish-green rock from the New Idria 200< velocity at the lowest velocities tested. We estimate that pure chrys- district, California. The rock is essentially all serpentine, and X-ray otile gouge at hydrostatic fluid pressure and appropriate temper- diffraction analysis combined with petrographic observations sug- atures would have shear strength averaged over a depth of 14 km gests the presence of clinochrysotile alone. The chrysotile has a 2ϩ of 50 MPa. Thus, on the sole basis of its strength, chrysotile cannot uniform composition of approximately Mg5.62Fe 0.30Al0.05 be the cause of a weak San Andreas fault. However, chrysotile may Si4O10(OH)8, calculated from electron microprobe analyses. The also contribute to low fault strength by forming mineral seals that rock was hand ground and passed through an 88-m-diameter sieve promote the development of high fluid pressures. to produce a simulated gouge. The strength tests were run in a triaxial machine fitted with an INTRODUCTION internal furnace. For each experiment, a 1-mm-thick layer of gouge The serpentine mineral chrysotile is commonly associated with was placed along a 30Њ sawcut in a 19.1-mm-diameter cylinder of faults and shear zones (e.g., O’Hanley, 1991). At several localities in antigoritic serpentinite. Serpentinite cylinders were used to avoid California, for example, the chrysotile content of sheared serpen- the possibility of reaction with the gouge during tests at elevated tinite is higher than that of nearby unsheared serpentinite (Page, temperatures. A borehole for pore-fluid entry was drilled into the 1968; Coleman and Keith, 1971; Mumpton and Thompson, 1975). upper half of each cylinder. The sample was contained in a copper Knowledge of the frictional properties and mode of occurrence of jacket during the experiment, to separate it from the confining- chrysotile may therefore be important to understanding the behav- pressure medium; reported strengths have been corrected for the ior of serpentinite-bearing sections of the San Andreas and related strength of the jacket. Selected runs, using polyurethane and viton faults. jackets, were conducted to verify the corrections for copper-jacket The absence of a significant conductive heat-flow anomaly di- strength. Confining pressure was applied first to the sample, fol- rectly over the San Andreas fault suggests, on the basis of models of lowed by the pore pressure. After the pressures had equilibrated, conductive heat transport, that the average frictional resistance in the temperature was raised to the desired value. Temperature was the seismogenic zone during fault motion is Յ20 MPa (Brune et al., monitored by a thermocouple inserted along the pore-pressure in- 1969; Lachenbruch and Sass, 1980). In addition, Mount and Suppe let. The experiments were all run at a constant normal stress, which (1987) and Zoback et al. (1987) determined that the maximum prin- was maintained by means of computer-controlled adjustments to cipal stress generally is almost perpendicular to the San Andreas the confining pressure. As a test of pore-fluid communication, at the fault. Fault-normal compression imposes additional limitations on end of two 200 ЊC experiments, pore pressure.and normal stress average fault strength, to the extent that if a hydrostatic fluid-pres- were increased together by 5 MPa, at a constant velocity of 0.2 m/s. sure gradient is assumed, the coefficient of friction, (ϭ shear In both cases, essentially no change in shear strength was measured, stress/[normal stress Ϫ pore pressure]), of fault-zone materials may as expected for a sample in good hydraulic communication with the need to be Յ0.1 to account for this weakness (Lachenbruch and external pore-pressure system. Had the fault been hydraulically iso- Sass, 1992). Previous strength investigations on serpentinite have lated from the pore-pressure system, the increase in normal stress concentrated on the antigorite- and lizardite-rich types, both of would have resulted in a 5% increase in shear strength. which are relatively strong (Raleigh and Paterson, 1965; Dengo and We conducted two groups of experiments for this study. (1) A Logan, 1981; Reinen et al., 1991). The strength of chrysotile has only series was run at 10 MPa pore pressure of deionized water and a recently been considered, but preliminary reports indicate that it constant normal stress of 110 MPa, to yield a constant effective may be less than half as strong as the other two varieties, averaging normal stress of 100 MPa. The experiments were run at tempera- Ϸ0.20–0.25 at room temperature (Reinen et al., 1993; note: tures of 25, 100, and 200 ЊC, with axial shortening rates stepped in Reinen et al., 1993, 1994, reported a low strength for a serpentinite the range 0.001 to 10 m/s. The slowest rate is equivalent to a sliding gouge that they originally identified as lizardite but that Reinen and velocity along the inclined gouge layer of 36.4 mm/yr, which is close Tullis, 1995, subsequently determined to be chrysotile). Further- to the average slip rate of the San Andreas fault in central Califor- Geology; November 1996; v. 24; no. 11; p. 1041–1044; 3 figures. 1041 nia. (2) A series was run at the temperature-pressure conditions samples yield the lowest and highest coefficients of friction, respec- corresponding to depths of 3, 6, and 9 km in the fault, assuming a tively, obtained for chrysotile in this study. At 290 ЊC, the coefficient hydrostatic pore-pressure gradient and a geothermal gradient of of friction exceeds 0.5 and is comparable to estimates of at 290 ЊC 30Њ/km (Lachenbruch and Sass, 1973). The 9 km simulation, con- for lizardite- and antigorite-rich serpentinite gouges at 290 ЊC, ex- ducted at 290 ЊC, is close to the upper temperature limit of stability trapolated from our data at 200 ЊC (Moore et al., 1995). The 200 and of chrysotile (Evans et al., 1976). At greater depths, chrysotile 290 ЊC samples show pronounced increases in during the 0.001 should be replaced by antigorite. Combined petrographic, X-ray m/s step, which occurs early in these experiments, and remains diffraction, and scanning electron microscope analyses of the sam- high for the rest of the experiments. Changing velocity has little ples yielded no evidence of chrysotile breakdown, to Ϸ1 m reso- effect on chrysotile strength at 200 and 290 ЊC. At 110 ЊC, is as low lution, during any of the experiments. as 0.1 at the slowest velocity steps, compared to a minimum of ϭ 0.15 for comparable velocities in the 100 ЊC experiment (Fig. 1B). RESULTS The 110 ЊC experiment was conducted at about one-half the effec- The low room-temperature strength of the chrysotile gouge tive normal stress of the 100 ЊC experiments, which suggests that (Ϸ0.20) measured at 100 MPa effective normal stress (Fig. 1) is for chrysotile may increase with increasing effective stress. In con- consistent with the values reported by Reinen et al. (1993). At the trast, the coefficient of friction of both lizardite and antigorite ser- faster rates of 0.1 to 10 m/s (Fig. 1A), the two heated samples are pentinite decreases with increasing effective pressure (e.g., Raleigh stronger overall than the one run at room temperature, although the and Paterson, 1965). We have planned a series of experiments to differences are slight. At lower velocities (Fig. 1B), however, the better separate the controls of temperature, effective stress, and 200 ЊC sample is significantly stronger, with approaching 0.35 fluid pressure on chrysotile strength. when the jacket failed. Much of this increase is attributable to a time-dependent hardening of the gouge during the interval at 0.001 DISCUSSION m/s; 0.5 mm axial displacement at that rate required nearly 5.8 days Our results corroborate the findings of Reinen et al. (1993) that to complete. at low temperatures chrysotile is only about half as strong as the Our room-temperature results (Fig. 1A) are consistent with the more abundant serpentine minerals lizardite and antigorite.
Details
-
File Typepdf
-
Upload Time-
-
Content LanguagesEnglish
-
Upload UserAnonymous/Not logged-in
-
File Pages4 Page
-
File Size-