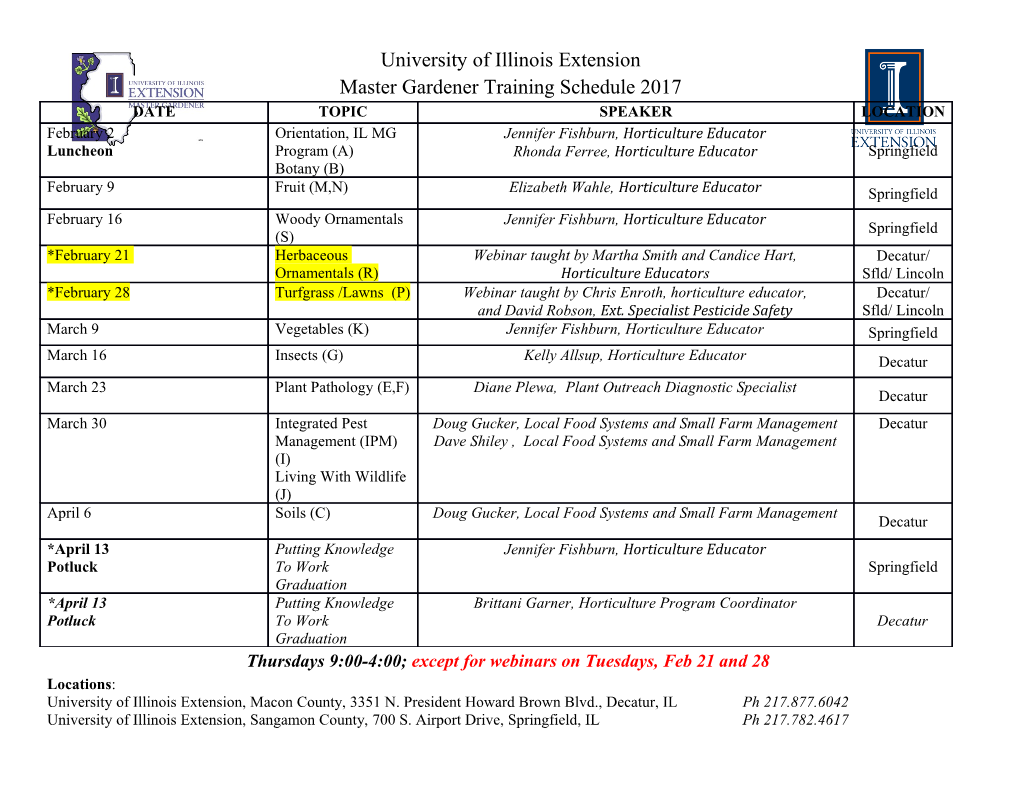
18.785 Number theory I Fall 2017 Lecture #5 09/20/2017 5 Dedekind extensions In this lecture we prove that the integral closure of a Dedekind domain in a finite extension of its fraction field is also a Dedekind domain; this implies, in particular, that the ring of integers of a number field is a Dedekind domain. We then consider the factorization of prime ideals in Dedekind extensions. 5.1 Dual modules, pairings, and lattices In this section we work in a more general setting, where A is any commutative (unital) ring. Definition 5.1. Let A be a commutative ring and M an A-module. The dual module M _ is the A-module HomA(M; A) with scalar multiplication (af)(m) = af(m), where a 2 A, f 2 HomA(M; A), and m 2 M. If ': M ! N is an A-module homomorphism, the dual homomorphism '_ : N _ ! M _ is defined by '_(g)(m) = g('(m)), for g 2 N _ and m 2 M. It is easy to check that taking duals preserves identity maps and is compatible with composition: if '1 : M ! N and '2 : N ! P are A-module homomorphisms, then _ _ _ ('2'1) = '1 '2 . We thus have a contravariant functor from the category of A-modules to itself. This functor is compatible with (finite) direct sums, (M ⊕ N)_ ' M _ ⊕ N _. Lemma 5.2. Let A be a commutative ring. For all A-modules M and N the A-modules (M ⊕ N)_ and M _ ⊕ N _ are canonically isomorphic. Proof. We have inverse A-module homomorphisms ' 7! (m 7! '(m; 0); n 7! '(0; n)) and (φ, ) 7! ((m; n) 7! φ(m) + (n)). We have already seen some examples of dual modules: each invertible fractional ideal is isomorphic to the dual of its inverse. Proposition 5.3. Let A be an integral domain with fraction field K and let M be a nonzero A-submodule of K. Then M _ ' (A : M) := fx 2 K : xM ⊆ Ag; in particular, if M is an invertible fractional ideal then M _ ' M −1 and M __ ' M. Proof. For any x 2 (A : M) the map m 7! xm is an A-linear map from M to A, hence an element of M _, and this defines an A-module homomorphism ':(A : M) ! M _, since the map x 7! (m 7! xm) is itself A-linear. Since M ⊆ K is a nonzero A-module, it contains some nonzero a 2 A (if a=b 2 M, so is ba=b = a). If f 2 M _ and m = b=c 2 M then b ac b b ac b f(a) f(m) = f = f = f = f(a) = m; c ac c ac c ac a where we have used the fact that a1f(a2=a3) = a2f(a1=a3) for any a1; a2; a3 2 A with a1=a3; a2=a3 2 M, by the A-linearity of f. It follows that f corresponds to multiplication by x = f(a)=a, which lies in (A : M) since xm = f(m) 2 A for all m 2 M. The map f 7! f(a)=a defines an A-module homomorphism M _ ! (A : M) inverse to ', so ' is an isomorphism. When M is an invertible fractional ideal we have M _ ' (A : M) = M −1, by Lemma 3.2, and M __ ' (M −1)−1 = M follows. If A is a field, then M is a vector space, M _ is its dual space and we have M __ ' M, as in the proposition above. But in general not every A-module is isomorphic to its double dual; those that are are said to be reflexive. Andrew V. Sutherland _ Example 5.4. As a Z-module, we have Q = f0g because there are no non-trivial Z-linear homomorphisms from Q to Z; indeed, Q is a divisible group and Z contains no non-trivial __ _ __ divisible subgroups. It follows that Q = f0g (but as Q-modules we have Q ' Q ' Q ). Similarly, the dual of any finite Z-module (any finite abelian group) is the zero module, as is the double dual. More generally, if A is an integral domain every dual (and double dual) A-module must be torsion free, but not all A-modules are torsion free. One situation where we can recover many of the standard results that hold for vector spaces of finite dimension (with essentially the same proofs), is when M is a free module of finite rank. In particular, not only is M reflexive, we have M ' M _ (non-canonically) and may explicitly construct a dual basis. Theorem 5.5. Let A be a commutative ring and let M be a free A-module of rank n. Then _ M is also a free A-module of rank n, and each basis (e1;:: : ; en) of M uniquely determines _ _ _ a dual basis (e1 ; : : : en) of M with the property ( _ 1 i = j; e (ej) = δij := i 0 i 6= j: Proof. If n = 0 then M = M _ = f0g and the theorem holds. Now assume n ≥ 1 and fix an n _ A-basis e := (e1;:: : ; en) for M. For each a := (a1; : : : ; an) 2 A , define fa 2 M by setting fa(ei) = ai and extending A-linearly. The map a 7! fa gives an A-module homomorphism n _ A ! M with inverse f 7! (f(e1); : : : ; f(en)) and is therefore an isomorphism. It follows that M _ ' An is a free A-module of rank n. _ n Now let ei := f^ι, where ^ι := (0;:::; 0; 1; 0;:::; 0) 2 A has a 1 in the ith position. Then _ _ _ _ ^ n _ e := (e1 ; : : : ; en) is a basis for M , since (1; : : : ; n^) is a basis for A , and ei (ej) = δij. The basis e_ is uniquely determined by e: it must be the image of (1^; : : : ; n^) under the isomorphism a 7! fa determined by e. Definition 5.6. Let A be a commutative ring and M an A-module. A (bilinear) pairing on M is an A-linear map h·; ·i: M × M ! A. Explicitly, this means that for all u; v; w 2 M and λ 2 A we have hu + v; wi = hu; wi + hv; wi; hu; v + wi = hu; vi + hu; wi; hλu, vi = hu; λvi = λhu; vi: If hv; wi = hw; vi then h·; ·i is symmetric, if hv; wi = −hw; vi then h·; ·i is skew-symmetric, and if hv; vi = 0 then h·; ·i is alternating (the last two are equivalent provided char(A) 6= 2). The pairing h·; ·i induces an A-module homomorphism ': M ! M _ m 7! (n 7! hm; ni) If ker ' = f0g then h·; ·i is nondegenerate, and if ' is an isomorphism then h·; ·i is perfect. Every perfect pairing is necessarily nondegenerate. If M is a vector space of finite dimension the converse holds, but this is not true in general, not even for free modules of finite rank: consider the pairing hx; yi := 2xy on Z, which is non-degenerate but not perfect. 18.785 Fall 2017, Lecture #5, Page 2 If M is a free A-module with basis (e1;::: ; en) and h·; ·i is a perfect pairing, we can apply the inverse of the isomorphism ': M −∼! M _ induced by the pairing to the dual _ _ 0 0 basis (e1 ; : : : ; en) given by Theorem 5.5 to obtain a basis (e1; : : : ; en) for M that satisfies 0 hei; eji = δij: 0 0 When h·; ·i is symmetric we can similarly recover (e1; : : : ; en) from (e1; : : : ; en) in the same way. We record this fact in the following proposition. Proposition 5.7. Let A be a commutative ring and let M be a free A-module of rank n with 0 0 a perfect pairing h·; ·i. For each A-basis (e1; : : : ; en) of M there is a unique basis (e1; : : : ; en) 0 for M such that hei; eji = δij. Proof. Existence follows from the discussion above: apply the inverse of the isomorphism _ _ _ ': V ! V induced by h·; ·i to the dual basis (e1 ; : : : ; en) given by Theorem 5.5 to obtain 0 0 0 −1 _ _ 0 0 a basis (e1; : : : ; en) for M with ei = ' (ei ). We then have ei = '(ei) = m 7! hei; mi and 0 0 _ hei; eji = '(ei)(ej) = ei (ej) = δij 0 0 for 1 ≤ i; j ≤ n. If (f1; : : : ; fn) is another basis for M with the same property then for 0 0 0 0 each i we have hei − fi ; eji = δij − δij = 0 for every ej, and therefore hei − fi ; mi = 0 for 0 0 all m 2 M, but then ei − fi 2 ker ' = f0g, since the perfect pairing h·; ·i is nondegenerate, 0 0 and therefore fi = ei for each i; uniqueness follows. Remark 5.8. In what follows the commutative ring A in Proposition 5.7 will typically be a field K and the free A-module M will be a K-vector space that we will denote V . We may then use A to denote a subring of K and M to denote an A-submodule of V .A perfect paring h·; ·i on the K-vector space V will typically not restrict to a perfect pairing on the A-module M. For example, the perfect pairing hx; yi = xy on Q does not restrict _ to a perfect pairing on the Z-module 2Z because the induced map ': 2Z ! 2Z defined by _ '(m) = (n 7! mn) is not surjective: the map x 7! x=2 lies in 2Z = HomZ(2Z; Z) but it is not in the image of '.
Details
-
File Typepdf
-
Upload Time-
-
Content LanguagesEnglish
-
Upload UserAnonymous/Not logged-in
-
File Pages13 Page
-
File Size-