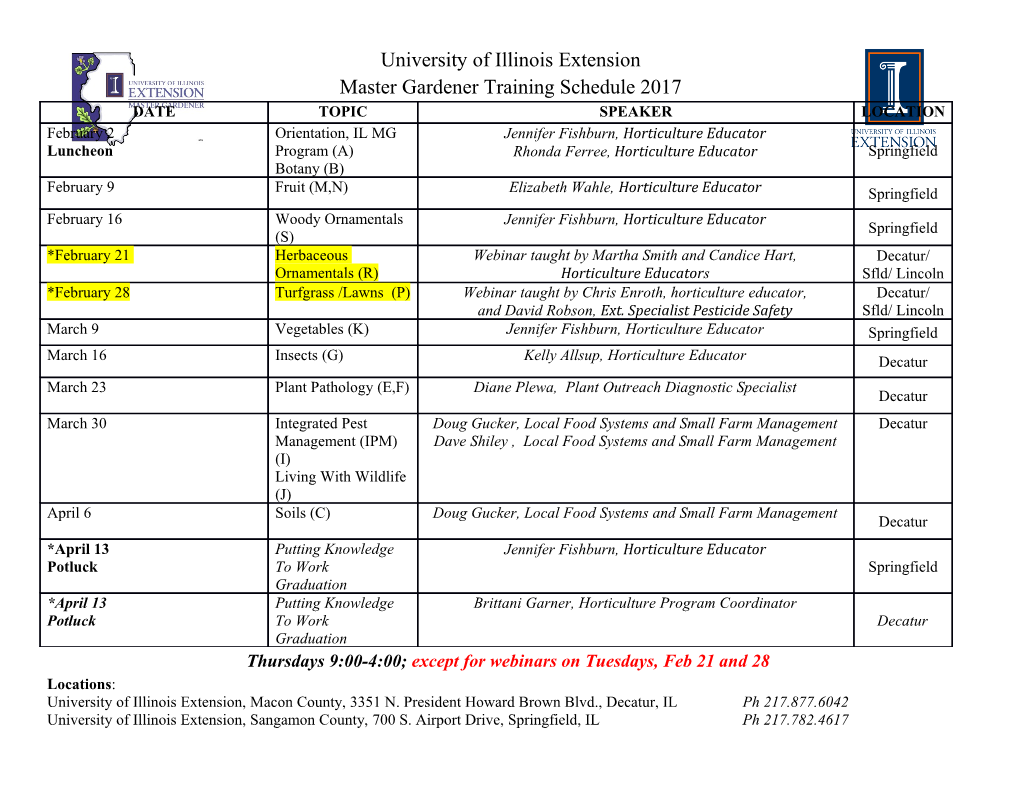
Earth and Planetary Science Letters 387 (2014) 27–33 Contents lists available at ScienceDirect Earth and Planetary Science Letters www.elsevier.com/locate/epsl The Mid-Pliocene sea-level conundrum: Glacial isostasy, eustasy and dynamic topography ∗ A. Rovere a, , M.E. Raymo a, J.X. Mitrovica b,P.J.Heartyc,M.J.O’Learyd,J.D.Inglise a Lamont Doherty Earth Observatory, Columbia University, 61 Route 9W, Palisades, NY 10964, USA b Department of Earth and Planetary Sciences, Harvard University, 20 Oxford Street, Cambridge, MA 02138, USA c Department of Environmental Studies, University of North Carolina at Wilmington, Wilmington, NC 28403, USA d Department of Environment and Agriculture, Curtin University, Bentley, Western Australia 6102, Australia e Department of Geological Sciences, University of North Carolina at Chapel Hill, Chapel Hill, NC 27516, United States article info abstract Article history: Determining eustatic sea level during the Mid-Pliocene warm period (∼3.3 to 2.9 Ma) has been a central Received 31 May 2013 but elusive goal in the study of past warm climates. Estimates of eustatic sea level based on geologic Received in revised form 7 October 2013 data span a broad range; variation that we now recognize is due in part to geographically varying Accepted 23 October 2013 post-depositional displacement caused by glacial isostatic adjustment and dynamic topography. In this Available online xxxx study, we combine field observations and glacial isostatic adjustment modeling to estimate the dynamic Editor: J. Lynch-Stieglitz topography signal in three areas that are important to paleo-sea level studies of the Mid-Pliocene warm Keywords: period (South Africa, West Australia and southeastern United States). We show that dynamic topography Mid-Pliocene sea level played a significant role in the post-depositional displacement of Pliocene, and even younger Pleistocene, Australia shorelines. In this regard, we provide a robust paleo-sea level elevation data set, corrected for glacial Republic of South Africa isostatic adjustment, that can be used to evaluate predictions from mantle flow models of dynamic southeast coast USA topography. dynamic topography © 2013 Elsevier B.V. All rights reserved. isostasy 1. Introduction sea-surface temperature), sea-level estimates for the MPWP vary widely, in part because signals associated with glacial isostatic ad- The Mid-Pliocene warm period (MPWP), historically defined justment (GIA) and dynamic topography (that is, topography sup- as the interval between ∼3.3 and 2.9 Ma, is widely considered ported by convectively-induced viscous stresses in the mantle and to be an example of a past climate state in equilibrium with associated buoyancy variations in the lithosphere, henceforth “DT”) ∼400 ppmv atmospheric CO2 levels (Pagani et al., 2009, compare have only recently been taken into account (Raymo et al., 2011; to ∼400 ppmv value as of May 2013; see Fig. S1, Supplementary Rowley et al., 2013). Materials, for a synopsis). For this reason, this interval of time is an Along several passive margins, Mid- to late-Pliocene shallow attractive target for climate model validation studies that compare water deposits are sometimes found tens of kilometers inland from model predictions with climate proxy reconstructions. However, for the present-day shoreline, often at the base of distinctive scarps one key climate variable, polar ice volume in the Pliocene rela- (Dowsett and Cronin, 1990; James et al., 2006). These scarps were tive to today, little consensus exists in the literature. An accurate carved, by the relentless erosive action of the sea over the course estimate of peak eustatic sea level (ESL) at this time would pro- of dozens of orbitally-paced SL highstands that occurred between vide insight into both the stability of Greenland and Antarctic ice the late Miocene and late Pliocene (e.g., Lisiecki and Raymo, 2005). sheets in a slightly warmer climate and help resolve discrepan- Further, the benthic oxygen isotope record (Lisiecki and Raymo, cies between ice sheet model predictions and data (e.g., Pollard 2005) suggests that these highstands all peaked approximately the and DeConto, 2009). (In this paper we define ESL change as the same eustatic value (see Fig. S1, Supplementary Materials, for de- geographically uniform change in sea level that would be equal tails). As the ocean eroded steadily inland carving the paleo-sea to the volume of meltwater flux into or out of the ocean.) De- cliffs, broad coastal terraces also evolved – these coastal plains spite the general agreement on other climatic variables (such as are now observed to be mantled with younger Pliocene and Pleis- tocene sediments. At the break in slope, also called the toe, or ‘inner margin,’ of the scarp, shallow marine deposits date to the * Corresponding author. Tel.: +1 347 416 1164. late Pliocene, a time correlative with the onset of global cooling as- E-mail address: [email protected] (A. Rovere). sociated with the intensification of northern hemisphere glaciation. 0012-821X/$ – see front matter © 2013 Elsevier B.V. All rights reserved. http://dx.doi.org/10.1016/j.epsl.2013.10.030 28 A. Rovere et al. / Earth and Planetary Science Letters 387 (2014) 27–33 Fig. 1. Geographic location and elevation plots of MPWP shorelines at (a) De Hoop, South Africa, (b) Roe Plain, Australia and (c) the southeast US coast. Small black dots represent the position of paleo SL obtained from inner margins of broad terrace surfaces measured on DEMs. Labeled blue dots represent paleo SL position obtained from RSL markers measured in the field (refer to Table 1 and Supplementary Material). The black dotted line connects all the paleo SLs obtained in one area. The gray band represents the standard deviation of paleo SL points. The blue and red dashed lines represent the position of the paleo SL after GIA correction based on the LM (blue)andVM2(red) viscosity models. In frame (b) the white lines on the map represent the main faults identified on the Nullabor and Roe Plains (Clark et al., 2012). In frame (c) the numbers 1–4 represent locations where the Duplin Formation has been sampled (corresponding to sites 1–4 of Dowsett and Cronin, 1990) while numbers 5–7 are locations of the Raysor Formation identified by Huddlestun (1988). Sr isotopic ratios yielded ages of 2.88–3.57 Ma for site 3 (McGregor et al., 2011), and of 2.3–2.8 Ma for site 4 (Graybill et al., 2009). Dashed areas in (b) and (c) represent the mapped extension of the Roe Calcarenite (James et al., 2006) as well as the Duplin Formation (SC) and Duplin–Yorktown Formation (Dicken et al., 2007). All distances in lower plots are calculated as linear distance from the beginning of the lines representing the scarp on the map. The projection used for the three maps is geographic coordinate system, WGS 84. (For interpretation of the references to color in this figure legend, the reader is referred to the web version of this article.) We interpret the slope break as indicative of the former level of described herein, we accounted for depositional effects due to GIA the sea just prior to the long-term global cooling trend that began using a large set of numerical modeling results. at ∼2.9 Ma (see Supplementary Materials for details). We then combine GIA-corrected scarp elevations with differ- Using digital elevation models (DEMs) and field surveys, we ent eustatic sea-level scenarios to calculate a set of field-based measured the elevation of the slope break of three such scarps, DT predictions for each area, and compare these with previously as well as associated SL markers, across hundreds of kilometers in published DT models. Our ultimate aim is to provide a robust the southern Republic of South Africa, southern Western Australia, dataset (attached as Supplementary Material) against which future and southeastern United States (Fig. 1a, b, c and Table 1). Then, as DT model predictions in these three areas can be tested. A. Rovere et al. / Earth and Planetary Science Letters 387 (2014) 27–33 29 Table 1 Sedimentary and paleo-biological facies of sites (blue dots in Fig. 1), located near the base of the scarp, measurement error (GPS vertical error), elevation of the scarp base measured near the site and calculation of the paleo SL elevation as represented in Fig. 1 (see Supplementary Materials for details). All measurements are in meters. Site Name Elevation of Scarp PaleoSLelevation Description marine facies elevation (as plotted in (marine limiting) (m) Fig. 1) (m) (m) ZBR1 Bredasdorp, 21.89 ± 0.222.52 ± 0.224.02 ± 1.51 Large marine Glycymeris and Ostrea, as described in earlier works on the South Africa Pliocene De Hoop formation16 are found near the base of the scarp. ZDH De Hoop, 26.27 ± 128.32 ± 129.82 ± 1.8 Coarse, pebbly, fossiliferous calcarenite with large disarticulated Glycymeris South Africa oriented concave down (shoaling) exposed in a near horizontal bench that regionally defines the top of the terrace surface. RMA1 Madura Quarry, 27.64 ± 0.68 29.3 ± 0.68 30.8 ± 1.65 Articulated shells of the venerid bivalve Katelysia,whosemodern Australia representatives live in a sandy littoral seabed17. REL1 Elarbilla, 28.44 ± 0.28 29.45 ± 0.28 30.95 ± 1.53 Fossil shells of Madra rufescens were sampled within a sandy matrix, Australia typical of a beach environment. As the shells were for most part concave down, this facies could represent an intertidal to shallow subtidal paleoenvironment. RCC1 Carlabeencabba, 17.50 ± 0.13 19.62 ± 0.13 21.12 ± 1.51 Mactra rufescens shells were sampled within a unit characterized by Australia borrows of vagile organisms, indicating a marine environment at this site. RBB1 Boolaboola, 18.99 ± 0.17 19.2 ± 0.17 20.7 ± 1.51 Marine Glycymeris radians shells were sampled within a unit characterized Australia by some articulated shells and several randomly oriented ones.
Details
-
File Typepdf
-
Upload Time-
-
Content LanguagesEnglish
-
Upload UserAnonymous/Not logged-in
-
File Pages7 Page
-
File Size-