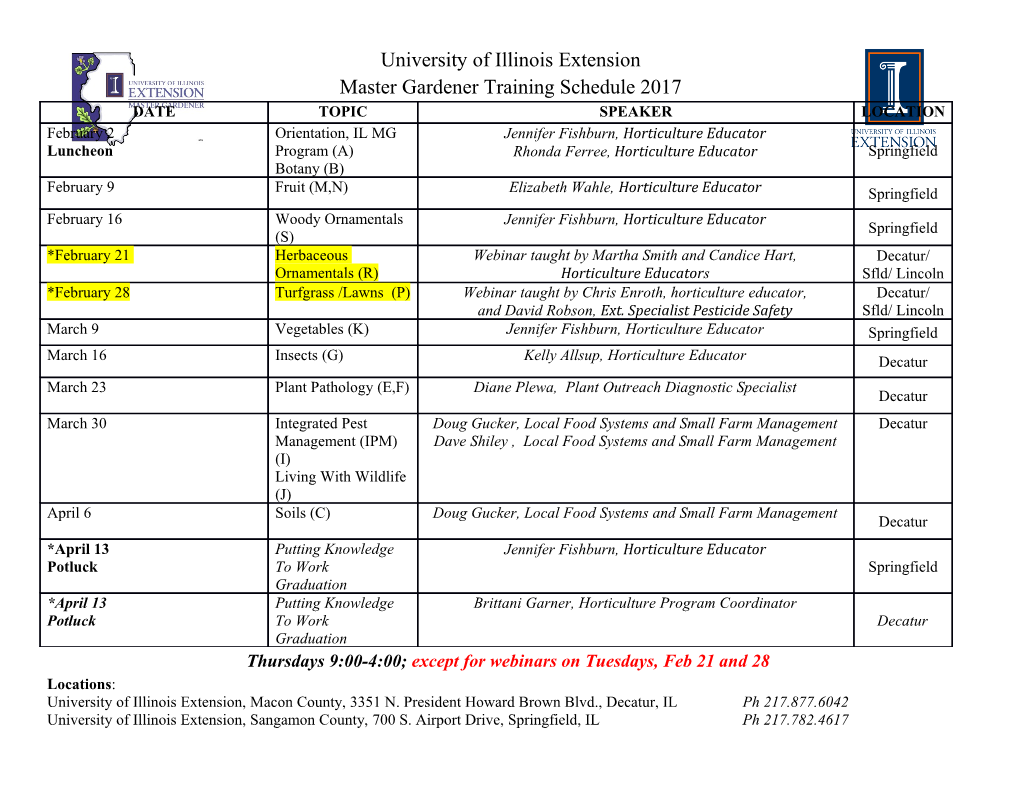
Definition: The residue Res(f, c) of a function f(z) at c is the coefficient of (z − c)−1 in the Laurent series expansion of f at c. Computation: To compute Res(f, c) , consider (z‐c)f(z) =: g(z) = g(c) + … +(z‐c)ng(n)(c)/n! +… . ‐1 n‐1 (n) Then f(z)=(z‐c) g(c) + … + (z‐c) g (c)/n! . Thus Res(f, c) = g(c) = (z‐c)f(z)|z=c . If f is analytical in a neighborhood of c, then Res(f, c) = (z‐c)f(z)|z=c = 0. The converse is not generally true. At a simple pole c, the residue of f is given by: More generally, if c is a pole of order n, then f(z)=h(z)/(z‐c)n, and so Res(f, c) is given by: n n‐1 (n‐1) n (n‐1) (z‐c)f(z)|z=c = (z‐c) h(z)/(z‐c) |z=c = h(z)/(z‐c) |z=c = h (z)/(n‐1)!|z=c =[(z‐c) f(z)] / (n‐1)!|z=c , where the 3rd equality is obtained by differentiating the numerator and denominator (n‐1) times. In other words, if c is a pole of order n, then residue of f at c can be determined by the formula: This formula can be very useful in determining the residues for low‐order poles. For higher order poles, the calculations can become unmanageable, and a direct series expansion may be easier. Application: According to residue theorem: th Here, γ is a closed‐curve, and I(γ, ak) counts the number of times γ winds around ak (k point inside the closed‐curve γ where f is not analytic) in a counter‐clockwise manner. Since residues can be computed quite easily (as discussed above), they can be used to easily determine a contour integral via the above residue theorem. As an example, the above theorem is used in deriving the Nyquist criterion for stability (#RHP poles of closed‐loop = #RHP poles of open‐loop + #encirclements of (‐1,0) by plot of open‐loop freq.‐response). The residue theorem and its applications Oliver Knill Caltech, 1996 This text contains some notes to a three hour lecture in complex analysis given at Caltech. The lectures start from scratch and contain an essentially self-contained proof of the Jordan normal form theorem, I had learned from Eugene Trubowitz as an undergraduate at ETH Z¨urich in the third semester of the standard calculus education at that school. My text also includes two proofs of the fundamental theorem of algebra using complex analysis and examples, which examples showing how residue calculus can help to calculate some definite integrals. Except for the proof of the normal form theorem, the material is contained in standard text books on complex analysis. The notes assume familiarity with partial derivatives and line integrals. I use Trubowitz approach to use Greens theorem to prove Cauchy’s theorem. [ When I had been an undergraduate, such a direct multivariable link was not in my complex analysis text books (Ahlfors for example does not mention Greens theorem in his book).] For the Jordan form section, some linear algebra knowledge is required. 1 The residue theorem Definition Let D C be open (every point in D has a small disc around it which still is in D). Denote by C1(D) the differentiable⊂ functions D C. This means that for f(z) = f(x + iy) = u(x + iy)+ iv(x + iy) the partial derivatives → ∂u ∂u ∂v ∂v , , , ∂x ∂y ∂x ∂y are continuous, real-valued functions on D. Definition. Let γ : (a,b) D be a differentiable curve in D. Define the complex line integral → b f(z) dz = f(γ(t)) γ˙ (t) dt Zγ Za · If z = x + iy, and f = u + iv, we have b f(z) dz = (ux˙ vy˙)+ i(uy˙ + vx˙) dt Zγ Za − The integral for piecewise differentiable curves γ is obtained by adding the integrals of the pieces. We always assume from now on that all curves γ are piecewise differentiable. Example. D = z < r with r> 1, γ : [0, k 2π] D,γ(t) = (cos(t), sin(t)), f(z)= zn with k N, n Z. {| | } · → ∈ ∈ k2π k2π zn dz = einteiti dt = ei(n+1)ti dt Zγ Z0 Z0 If n = 1, we get 6 − n 1 i(n+2)t k2π z dz = e 0 =0 Zγ n +1 | n k2π If n = 1, we have γ z dz = 0 i dt = k 2πi. − R R · We recall from vector calculus the Green formula for a vector field (u, v) in R2 (ux˙ + vy˙) dt = (vx uy) dx dy , Zγ ZD − ∧ where D is the open set enclosed by closed curve γ = δD and where dx dy = dxdy is the volume form in the plane. Write dz = dx + idy, dz = dx idy and dz dz =2idx dy. Define∧ for f C1(D) − ∧ ∧ ∈ Theorem 1.1 (Complex Green Formula) f C1(D), D C, γ = δD. ∈ ⊂ ∂f f(z)dz = dz dz . Zγ ZD ∂z ∧ Proof. Green’s theorem applied twice (to the real part with the vector field (u, v) and to the imaginary part with the vector field (v,u)) shows that − b f(z) dz = (ux˙ vy˙)+ i (uy˙ + vx˙) dt Zγ Za − · coincides with ∂f 1 i dz dz = (ux vy)+ (uy + vx)2idx dy ZD ∂z ∧ ZD 2 − 2 ∧ = ( uy vx)dx dy + i (ux vy)dx dy ZD − − ∧ · ZD − ∧ We check that ∂f ∂u ∂v ∂v ∂u =0 = , = . ∂z ⇔ ∂x ∂y ∂x − ∂y The right hand side are called the Cauchy-Riemann differential equations. ω 1 ∂f ω Definition. Denote by C (D) the set of functions in C (D) for which ∂z = 0 for all z D. Functions f C (D) are called analytic or holomorphic in D. ∈ ∈ Corollary 1.2 (Theorem of Cauchy) f Cω(D), D C, γ = δD. ∈ ⊂ f(z) dz =0 . Zγ Proof. ∂f f(z) dz = dz dz =0 Zγ ZD ∂z ∧ Corollary 1.3 (Cauchy’s Integral formula) f Cω(D), D simply connected, γ = δD. For any a D ∈ ∈ 1 f(w) dw f(a)= . 2πi Z w a γ − it Proof. Define for small enough ǫ> 0 the set Dǫ = D z a ǫ and the curve γǫ : t z + ǫe . (Because D is open, the set D is contained in D for small enough ǫ\). {| Because− |≤γ } γ = δD we get by7→ Cauchy’s theorem 1.2 ǫ ∪− ǫ ǫ f(w) f(w) dw dw =0 . Z w a − Z w a γ − γǫ − We compute f(w) 2π f(z + ǫ eit) d 2π dw = · (a + ǫeit) dt = i f(a + ǫ eit) dt Z w a Z a + ǫ eit a dt · Z · γǫ − 0 · − 0 The right hand side converges for ǫ 0 to 2πif(a) because f C1(D) implies → ∈ f(a + ǫeit) f(a) C ǫ | − |≤ · Corollary 1.4 (Generalized Cauchy Integral formulas) Assume f Cω(D) and D C simply connected, and δD = γ. For all n N one has f (n∈)(z) Cω(D) and⊂ for any z / γ ∈ ∈ ∈ n! f(w) dz f (n)(z)= . 2πi Z (w z)n+1 γ − Proof. Just differentiate Cauchy’s integral formula n times. It follows that f Cω(D) is arbitrary often differentiable. ∈ Definition Let f Cω(D a ) and a D with simply connected D C with boundary γ. Define the residue of f at a as ∈ \{ } ∈ ⊂ 1 Res(f,a) := f(z) dz . 2πi Zγ By Cauchy’s theorem, the value does not depend on D. Example. f(z) = (z a)−1 and D = z a < 1 . Our calculation in the example at the beginning of the section gives Res(f,a)=1. − {| − | } A generalization of Cauchy’s theorem is the following residue theorem: Corollary 1.5 (The residue theorem) f Cω(D z n ), D open containing z with boundary δD = γ. ∈ \{ i}i=1 { i} 1 n f(z) dz = Res(f,z ) . 2πi Z i γ Xi=1 Proof. Take ǫ so small that Di = z zi ǫ are all disjoint and contained in D. Applying Cauchy’s theorem to the domain D n D leads to{| the− above|≤ formula.} \ 1=1 i S 2 Calculation of definite integrals The residue theorem has applications in functional analysis, linear algebra, analytic number theory, quantum field theory, algebraic geometry, Abelian integrals or dynamical systems. In this section we want to see how the residue theorem can be used to computing definite real integrals. The first example is the integral-sine x sin(t) Si(x)= dt , Z0 t a function which has applications in electrical engineering. It is used also in the proof of the prime number theorem which states that the function π(n)= p n p prime satisfies π(n) x/log(x) for x . { ≤ | } ∼ → ∞ ∞ sin(x) π Si( )= dx = ∞ Z0 x 2 eiz ω sin(x) Proof. Let f(z) = z which satisfies f C (C 0 ). For z = x R, we have Im(f(z)) = x . Define for ∈ \{ }4 ∈ R>ǫ> 0 the open set D enclosed by the curve γ = i=1 γi, where S Figure 1 γ1 : t [ǫ, R] t +0 i. γ : t ∈ [0, π] 7→ R eit·. 2 ∈ 7→ · γ3 : t [ R, ǫ] t +0 i. γ : t ∈ [π,− 0] − ǫ7→eit. · 4 ∈ 7→ · -R R By Cauchy’s theorem R ix π iReit ǫ ix 0 iǫeit e e it e e it 0= f(z) dz = dx + it iRe dt + dx + it iǫe dt .
Details
-
File Typepdf
-
Upload Time-
-
Content LanguagesEnglish
-
Upload UserAnonymous/Not logged-in
-
File Pages11 Page
-
File Size-