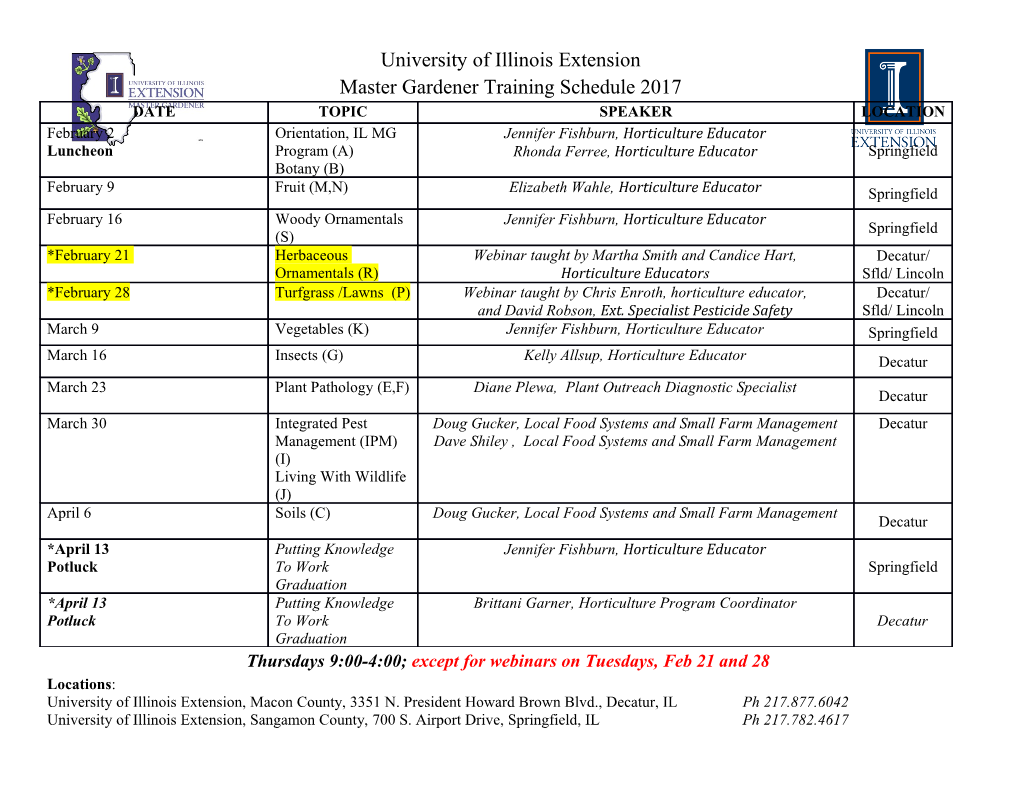
INTERNAL ARCHITECTURE OF THE PROTO- KERN CANYON FAULT AT ENGINEER’S POINT, LAKE ISABELLA, CALIFORNIA By Zachary Scott Martindale, B.S. A Thesis Submitted to the Department of Geological Sciences California State University, Bakersfield In Partial Fulfillment for the Degree of Masters of Science in Geology Spring 2015 Copyright By Zachary Scott Martindale 2015 Acknowledgements Completion of this project would not have been possible without the generous funding from CSUB’s NSF CREST program, the Student Research Scholarship program, and the Graduate Student Center’s Grad Student Faculty Collaborative Initiative. I thank Dr. Negrini and Andrea Medina with the CSUB CREST program for their assistance in administrative matters. Numerous individuals have dedicated their time and patience in instructing me in operating various lab equipment: Kellie Townsend and Dr. Keith Putirka at Fresno State University; Alyssa Kaess, Kelsey Padilla, and Elizabeth Powers at CSUB. I also want to thank those who helped with advice in techniques of collection and interpretation of data: Drs. Sarah Brown, Junhua Guo, Robert Horton, and Chris Krugh. My deepest gratitude and respect is held for my advisor, Dr. Graham Andrews. His faith in me to accomplish a project of this magnitude was at times the only force pushing me forward. I would not have known how to begin any new stage without his direction. 1 Abstract Faults create a structurally weak zone within the lithosphere that can be reactivated again and again through geologic time. This reactivation can occur under varying tectonic stresses, temperatures, and pressures within the fault zone. This study focuses on the complex Kern Canyon Fault zone at Engineer’s Point, Lake Isabella, California, and begins to elucidate the geologic history recorded in its structure and alteration through the damage zone and fault core. The data suggest that the Miocene - Quaternary Kern Canyon Fault is hosted within a Cretaceous ductile shear zone and hydrothermal alteration zone, the proto-Kern Canyon shear zone. The proto-Kern Canyon shear zone is heavily and complexly fractured, and probably experienced dextral shear before the cessation of shearing and the onset of exhumation. The shear zone is thoroughly hydrothermally altered and has experienced phyllic and argillic alteration during and after deformation, respectively. The Kern Canyon Fault is located within this complexly fractured and altered bedrock; estimates of future seismicity should consider propensity for creep as well as stick-slip behavior. 2 Table of Contents List of Tables 4 List of Figures 5 Introduction 8 Methods 14 Results 17 Interpretation and Discussion 23 Summary 29 References 30 Tables 35 Figures 38 3 List of Tables Table 1: Calculated intensity and relative abundance values Table 2: Weight percentages of major elements by X-ray fluorescence Table 3: Trace element abundance by X-ray fluorescence 4 List of Figures Figure 1: Fault zone architecture with protolith, damage zone and fault core. From Caine et al. (1996). Figure 2: Table showing differentiated fault rock types. Modified from Sibson (1977). Figure 3: Fault zone architecture with depth. From Fossen (2010). Figure 4: Map of major faulting within south-central California. Figure 5: Earthquake epicenters around the Kern Canyon fault and the junction between the San Andreas and Garlock faults. Figure 6: Sketch map of Engineer’s Point showing locations of sample sites for analyses. Figure 7: Table of analyses conducted at each site. Figure 8: Map of fault rock lithologies and interpreted structures. Figure 9: Various structures and contacts within the map area. Figure 10: Map of fault rock lithologies in Domain 2. Figure 11: Photograph of Fractures within site Z01. Figure 12: Photograph of Fractures within site Z03. Figure 13: Photograph of fractures within site Z05. Figure 14: Photograph of fractures within site Z06. Figure 15: Photograph of fractures within site Z07. Figure 16: Photograph of fractures spanning Z08-Z10. Figure 17: Table showing summary of fracture data (stereonets, rose diagrams, fracture length distribution, fracture density) and interpretation for each station. Figure 18: Fracture length distribution within each zone. Figure 19: Rose diagrams of fracture orientations by zone. Figure 19: Poles to all fractures within each zone grouped by preferred orientation. Figure 20: Fracture lengths by zone on a log-log scale. 5 Figure 21: Poles to fractures in each zone grouped by orientation. Figure 22: Histogram of filled fractures by length and zone. Figure 23: Stereonets of poles to fractures separated by length. Figure 24: Stereonets of poles to fractures separated by fill. Figure 23: Poles to fractures separated by fill and length. Figure 26: Grain size distribution of clasts >1mm within the fault gouge of Z09 and Z10. Figure 27: Grain size distribution and statistics for Z08 grains <5 mm. Figure 28: Grain size distribution and statistics for Z09 grains <5 mm. Figure 29: Grain size distribution and statistics for Z10 grains <5 mm. Figure 28: Log-log plot of Z08, Z09 and Z10 grain size distribution and linear fits where possible. Figure 29: XRD patterns from 7° to 75° and interpreted mineralogy for each site. Figure 30: Detailed XRD patterns for each site. Figure 31: Compilation of XRD patterns for all sites to highlight trends through the fault zone. Figure 32: Abundances of major minerals relative only to that particular site. Figure 33: Abundances of major minerals relative to abundance in Z00 for each of the other sites. Figure 34: Clay to plagioclase ratio through the fault zone. Relative abundance is fairly linear except in Z08 and Z10b. Figure 37: Plot of major element/zirconium and selected trace element/ zirconium ratios for each station relative to Z00. Figure 38: Plot traced element abundances for each station relative to Z00. Figure 39: Table of hydrothermal alterations observed in each zone. Figure 40: Phyllic alterations in Z01. Figure 41: Argillic alteration in Z07. 6 Figure 42: Alteration of chlorite in Z02. Figure 43: Fractured oligoclase in Z00. Figure 44: Folded, fractured and altered chlorite. Figure 45: Gouge vein suggesting pulverization of calcite vein. Figure 46: Cataclasite with two domains of grain sizes. Figure 47: Increased magnification of cataclasite. Figure 48: In situ cataclasis of quartz and calcite. Figure 49: Multiple veins filling one fracture. Figure 50: Table of alterations within each zone. Figure 51: Waldron (2007) model of sheared fractures. Figure 52: Stereonet plots of preferred fracture orientations by zone showing rotation. 7 Introduction Faults are not discrete planes through the lithosphere. They are instead complex zones of deformation that can vary in type and degree (e.g., Engelder, 1974; Sibson, 1977; Caine et al., 1996). These variations include lithology, structure, alteration and grain size. All are heavily influenced by the presence of fluids; particularly ions within those fluids. Because varying stress regimes induce different structural deformations and the ions within fluids can vary throughout time, it may be possible to work out a deformation history for faults that have been reactivated numerous times in the past. This study analyzes and quantifies several of the variations that occur through the Kern Canyon fault zone, a long-lived and lengthy fault, along a 180 meter transect on the western side of the fault exposed at Engineer’s Point, Lake Isabella, California. Fault Zone Architecture The architecture of major fault zones and the deformation and hydrothermal alteration associated with them is often complex; however, development and evolution of such features dictate fault behavior. For example, in fracture mechanics the generation of new brittle faults and ”stick-slip” fault behavior is favored in isotropic, minimally fractured, and strong (i.e. non- hydrothermally altered) lithologies. Conversely, extensive fracture and foliation development favors reactivation of slip planes by ‘creep’ (i.e. Byerlee’s Law). Moreover, weakening of rocks by alteration (hydration, clay formation, etc.) favors creep through diffuse ductile deformation. Every fault zone is composed of three major zones: (1) the wall-rock protolith, (2) the damage zone, and (3) the fault core (Fig. 1; Chester and Logan, 1986; Goddard and Evans, 1995; Caine et al., 1996). The boundaries between each of these zones strike approximately parallel to the fault plane. The fault core contains the fault plane and accommodates the majority of the strain and alteration associated with the fault zone (Goddard and Evans, 1995; Caine et al., 1996). The damage zone envelopes the core and is composed of fractured, folded, cleaved and faulted rock that accommodate some significant portion of the shear stress (Caine et al., 1996). The protolith is the undamaged and unaltered rock that envelopes the damage zone. The width of each of these zones is dependent upon numerous factors including the host rock lithology, the direction and amount of relative offset, the amount of time over which offset has occurred, and the presence of fluid within the deformed fault rocks. (Goddard and Evans, 1995; Schulz and Evans, 2000). Progressively thicker zones are favored by protracted or large magnitude strain and hydrothermal alteration. A variety of different fault rock types exists (Fig. 2; Sibson, 1977). First order differentiations begin with determining whether the rock is cohesive or incohesive. Incohesive rocks are described based upon grain size as either breccia or gouge. Cohesive and incohesive rocks that exhibit grain size reduction but little to no shear or grain rounding are referred to as pulverized rocks (Mitchell et al., 2011). Cohesive rocks with a higher percentage of matrix are either mylonites, which have a distinct foliation, or cataclasites, with randomly oriented clasts. 8 The occurrence of foliation is closely linked to the pressure and temperature and therefore depth at which deformation took place. Typically, only brittle deformation occurs in the upper 10 km of the crust (Fig. 3). This brittle deformation results in the formation of fault breccias, pulverized rock, and gouge (Sibson, 1977).
Details
-
File Typepdf
-
Upload Time-
-
Content LanguagesEnglish
-
Upload UserAnonymous/Not logged-in
-
File Pages91 Page
-
File Size-