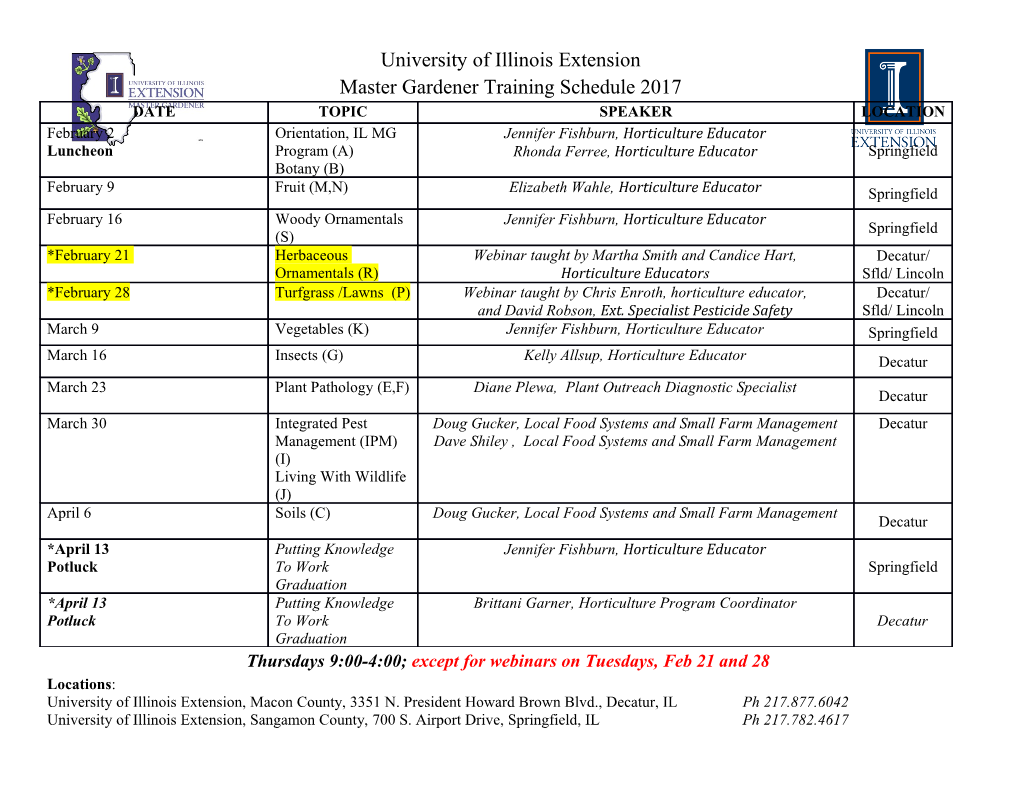
Please consider the environment before printing this thesis. This document is optimized for duplex printing. Telomere length of kakapo and other New Zealand birds - assessment of methods and applications A thesis submitted in partial fulfilment of the requirements for the degree of PhD in Cellular and Molecular Biology at the University of Canterbury by Thorsten Horn University of Canterbury 2008 Abstract Abstract The age structure of populations is an important and often unresolved factor in ecology and wildlife management. Parameters like onset of reproduction and senescence, reproductive success and survival rate are tightly correlated with age. Unfortunately, age information of wild animals is not easy to obtain, especially for birds, where few anatomical markers of age exist. Longitudinal age data from birds banded as chicks are rare, particularly in long lived species. Age estimation in such species would be extremely useful as their long life span typically indicates slow population growth and potentially the need for protection and conservation. Telomere length change has been suggested as a universal marker for ageing vertebrates and potentially other animals. This method, termed molecular ageing, is based on a shortening of telomeres with each cell division. In birds, the telomere length of erythrocytes has been reported to decline with age, as the founder cells (haematopoietic stem cells) divide to renew circulating red blood cells. I measured telomere length in kakapo, the world largest parrot and four other bird species (Buller’s albatross, kea, New Zealand robin and saddleback) using telomere restriction fragment analysis (TRF) to assess the potential for molecular ageing in these species. After providing an overview of methods to measure telomere length, I describe how one of them (TRF) measures telomere length by quantifying the size distribution of terminal restriction fragments using southern blot of in-gel hybridization (Chapter 2). Although TRF is currently the ‘gold standard’ to measure telomere length, it suffers from various technical problems that can compromise precision and accuracy of telomere length estimation. In addition, there are many variations of the protocol, complicating comparisons between publications. I focused on TRF analysis using a non-radioactive probe, because it does not require special precautions associated with handling and disposing of radioactive material and therefore is more suitable for ecology laboratories that typically do not have a strong molecular biology infrastructure. However, most of my findings can be applied to both, radioactive and non- radioactive TRF variants. I tested how sample storage, choice of restriction enzyme, gel I Abstract electrophoresis and choice of hybridization buffer can influence the results. Finally, I show how image analysis (e.g. background correction, gel calibration, formula to calculate telomere length and the analysis window) can not only change the magnitude of estimated telomere length, but also their correlation to each other. Based on these findings, I present and discuss an extensive list of methodological difficulties associated with TRF and present a protocol to obtain reliable and reproducible results. Using this optimized protocol, I then measured telomere length of 68 kakapo (Chapter 3). Almost half of the current kakapo population consists of birds that were captured as adults, hence only their minimum age is known (i.e. time from when they were found +5 years to reach adulthood). Although molecular ageing might not be able to predict chronological age accurately, as calibrated with minimum age of some birds, it should be able to compare relative age between birds. Recently, the oldest kakapo (Richard Henry) was found to show signs of reproductive senescence. The age (or telomere length) difference to Richard Henry could have been used to approximate the remaining reproductive time span for other birds. Unfortunately, there was no change of telomere length with age in cross sectional and longitudinal samples. Analysis of fitness data available for kakapo yielded correlations between telomere length and fledging success, but they were weak and disappeared when the most influential bird was excluded from analysis. The heavy management and small numbers of kakapo make conclusions about fitness and telomere length difficult and highly speculative. However, telomere length of mothers and their chicks were significantly correlated, a phenomena not previously observed in any bird. To test if the lack of telomere loss with age is specific to kakapo, I measured telomere length of one of its closest relatives: the kea (Chapter 4). Like kakapo, telomere length did not show any correlation with age. I then further assessed the usefulness of molecular ageing in birds using only chicks and very old birds to estimate the maximum TL range in an additional long lived (Buller’s albatross) and two shorter lived species (NZ robin and saddleback). In these II Abstract species, telomere length was on average higher in chicks than in adults. However, age matched individuals showed high variations in telomere length, such that age dependent and independent telomere length could not be distinguished. These data and published results from other bird species, coupled with the limitations of methodology I have identified (Chapter 2), indicate that molecular ageing does not work in most (if not all) birds in its current suggested form. Another way to measure telomere length is telomere Q-PCR, a real-time PCR based method. Measurement of the same kakapo samples with TRF and Q-PCR did not result in comparable results (Chapter 4). Through experimentation I found that differences in amplification efficiency between samples lead to unreliable estimation of telomere length using telomere Q-PCR. These differences were caused by inhibitors present in the samples. The problem of differential amplification efficiency in Q-PCR, while known, is largely ignored by the scientific community. Although some methods have been suggested to correct for differing efficiency, most of these introduce more error than they eliminate. I developed and applied an assay based on internal standard oligonucleotides that was able to corrected EDTA induced quantification errors of up to 70% with high precision and accuracy (Chapter 5). The method, however, failed when tested with other inhibitors commonly found in DNA samples extracted from blood (i.e. SDS, heparin, urea and FeCl3). PCR inhibition was highly selective in the probe-polymerase system I used, inhibiting amplification of genomic DNA, but not amplification of internal oligonucleotide or plasmid standards in the same reaction. Internal standards are a key feature of most diagnostic PCR assays to identify false negatives arising from amplification inhibition. The differential response to inhibition I identified greatly compromises the accuracy of these assays. Consequently, I strongly recommend that researchers using PCR assays with internal standards should verify that the target DNA and internal standard actually respond similarly to common inhibitors. III Acknowledgement Acknowledgement I would like to thank Professor Neil J. Gemmell for giving me the opportunity to conduct this thesis under his supervision and for providing funding for my money-consuming southern blots. I am especially grateful to Dr. Bruce C. (FS) Robertson for supervision (you google the best) and advice in the laboratory (to reduce the costs for southern blots). Also all the best to his family in their new home. Probably the biggest achievement of my thesis period is that I met Iris Jentzsch. I am deeply grateful for your love and support during this work (when Southern blots went wrong). I am looking forward to our shared future. I am indebted to Jonci Wolff for constructive smoke breaks (quit smoking, man !), being a good neighbour in the lab and for his contagious relaxedness, and to Margee Will for late night fries (Signature Range, RIP) and her contagious unrelaxedness. Many thanks also to Wiebke Müller for overwhelming discussions and statistical advice and Andrew Bagshaw for late night encounters and nasty plasmid preps. I would like to acknowledge our lab technicians Linda Morris, Joanne Burke for keeping the lab going and especially Maggie Tisch and her husband for adopting our fish. A special thanks to the New Zealand Department of Conservation (DOC) for support of the kakapo work, especially Daryl Eason, Graeme Elliott and the KPOs at Codfish Island. Additional bird samples were provided by the following: saddleback and NZ robin by Dr. Tania King and Dr. Ian Jamieson (University of Otago, NZ); zebra finch by Dr. Mark Hauber (University of Auckland, NZ); Buller’s albatross by Paul Sagar (NIWA, NZ) and Aaron Russ (University of Canterbury, NZ) and kea by Dr. Gyula Gajdon (Universität Wien, Austria). European sea bass data used for TRF optimization were collected at the IFREMER aquaculture station, Palavas-Les-Flots, France and supported by the European Community through the ‘Access to research infrastructures action of the improving human potential IV Acknowledgement programme HPRI-CT-2001 00146’ and through Bluefin Tuna in Captivity (REPRODOTT) Contract Nr.Q5RS 2002-01355. Many thanks to Professor C. R. Bridges (Heinrich-Heine- Universität Düsseldorf, Germany) for giving me the opportunity for this project. I thank the University of Canterbury and Landcare Research Sustaining and Restoring Biodiversity OBI for financial support of this
Details
-
File Typepdf
-
Upload Time-
-
Content LanguagesEnglish
-
Upload UserAnonymous/Not logged-in
-
File Pages237 Page
-
File Size-