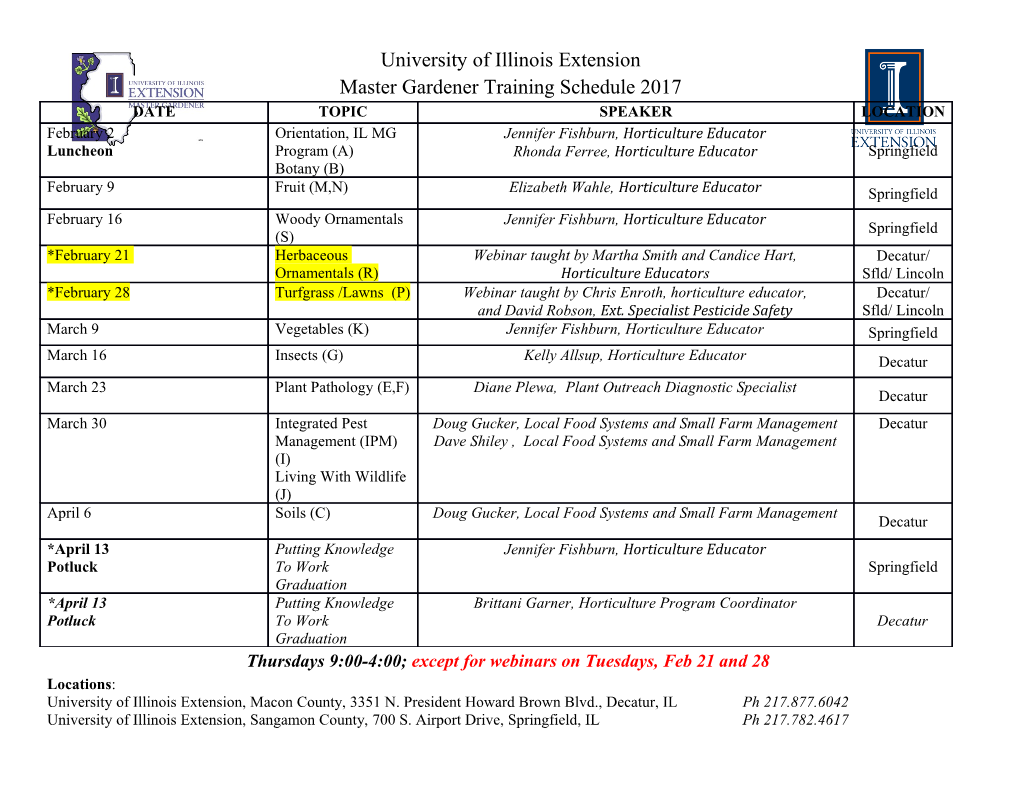
PERSPECTIVE PUBLISHED ONLINE: 10 APRIL 2017 | DOI: 10.1038/NGEO2922 The cold and relatively dry nature of mantle forearcs in subduction zones G. A. Abers1*, P. E. van Keken2 and B. R. Hacker3 Some of Earth’s coldest mantle is found in subduction zones at the tip of the mantle wedge that lies between the subducting and overriding plates. This forearc mantle is isolated from the flow of hot material beneath the volcanic arc, and so is inferred to reach temperatures no more than 600 to 800 °C — conditions at which hydrous mantle minerals should be stable. The forearc mantle could therefore constitute a significant reservoir for water if sufficient water is released from the subducting slab into the mantle wedge. Such a reservoir could hydrate the plate interface and has been invoked to aid the genesis of megathrust earthquakes and slow slip events. Our synthesis of results from thermal models that simulate the conditions for subduction zones globally, however, indicates that dehydration of subducting plates is too slow over the life span of a typical subduction zone to hydrate the forearc mantle. Hot subduction zones, where slabs dehydrate rapidly, are an exception. The hottest, most buoyant forearcs are most likely to survive plate collisions and be exhumed to the surface, so probably dominate the metamor- phic rock record. Analysis of global seismic data confirms the generally dry nature of mantle forearcs. We conclude that many subduction zones probably liberate insufficient water to hydrate the shallower plate boundary where great earthquakes and slow slip events nucleate. Thus, we suggest that it is solid-state processes and not hydration that leads to weakening of the plate interface in cold subduction zones. ubduction zones produce voluminous mantle-sourced mag- Arc 20 mas. This requires that the mantle temperatures below volcanic Upper-plate crust 300 1 arcs are sufficiently high for melting of hydrated peridotite . 600 Input flux S 40 Upper-plate Moho D 300 However, low heat flow and seismic attenuation indicate that the Cold wedge C shallow parts of mantle forearcs are cold and cannot be engaged A B 600 60 Forearc Slab in the mantle circulation beneath arcs. These data require a deep Hot flux decoupling between the top of the subducted plate and the over- wedge 900 2–4 80 Decoupling riding mantle wedge to depths of approximately 80 km . Hydrous Depth (km) Sub-arc st limit (D80) minerals such as chlorite and serpentine are stable in this cold flux u 1200 cr 100 g mantle forearc (Fig. 1). Low temperature alone is necessary but not in ct u d sufficient for hydrous phases to be present, because enough H2O b 120 u S needs to be available as well. Large H2O fluxes are required for full hydration, because serpentinites hold 12–13 wt% H O (ref. 5). 250 200 150 100 2 Distance from trench (km) Forearc H2O storage capacity Figure 1 | Schematic forearc showing water fluxes and potentially We estimate the potential for hydration of the cold forearc mantle hydrated mantle. Upper-plate mantle shows hydration variation: subarc wedge for a global suite of 56 subduction zone thermal and pet- anhydrous mantle (lherzolite) (A); potentially hydrated cold forearc 4,6,7 rological models by comparing the H2O flux from the slab pre- (B)–(D). The facies for hydrated lherzolite are: chlorite-talc lherzolite (B); dicted from those models with the mass of H2O that could be stored serpentine-chlorite wherlite (C); and serpentine-chlorite-brucite wherlite in the cold forearc mantle. To reproduce the ubiquitous observation (D) (see Supplementary Discussion 4). The grey shades indicate of low forearc heat flow and low seismic attenuation, these models temperature, labelled every 300 °C, whereas the red arrows and labels decouple the subducting slab from the overlying mantle to a depth show major water fluxes mediated by subduction. of 80 km. At greater depths, the slab is viscously coupled to the man- tle wedge driving the flow of hot asthenosphere below the volcanic arc3,4. Tests with 70 and 100 km decoupling depths show similar pat- (DMM)7,8. Mineral assemblages at each temperature and pressure terns and negligibly different results (see Supplementary Discussion are calculated for this composition via the Gibbs free energy mini- 4 for details and sensitivity tests). As a consequence, the slab surface mization program Perple_X9 using the methods and phase assem- transitions from <500 °C at <80 km depth to >800 °C at ~100 km blages detailed in ref. 7; Fig. 2b shows an example of the resulting (Fig. 2a), which is the typical depth of the slab below the volcanic H2O content. The same approach is used to estimate devolatilization arc. For the overlying mantle wedge we estimate the maximum pos- of the subducting sediment, crust and mantle, which provides esti- 6 sible mineral-bound water content for a water-saturated peridotite mates of H2O fluxes from the slab (Fig. 2e,f). These devolatilization of composition depleted mid-ocean ridge basalt (MORB) mantle models assume that all pore fluids are expelled at shallow depth. 1Department of Earth and Atmospheric Sciences, Cornell University, 2122 Snee Hall, Ithaca, New York 14853, USA. 2Department of Terrestrial Magnetism, Carnegie Institution for Science, 5241 Broad Branch Road NW, Washington DC 20015, USA. 3Department of Earth Science, University of California, Santa Barbara, Santa Barbara, California 93106, USA. *e-mail: [email protected] NATURE GEOSCIENCE | ADVANCE ONLINE PUBLICATION | www.nature.com/naturegeoscience 1 ©2017 Mac millan Publishers Li mited, part of Spri nger Nature. All ri ghts reserved. PERSPECTIVE NATURE GEOSCIENCE DOI: 10.1038/NGEO2922 a b Temperature T (°C) H2O capacity 1,400 20 20 1,200 kg m–3 40 40 300 1,000 250 60 400 60 1200 800 800 200 400 80 600 80 150 Depth (km) 100 100 800 400 100 50 200 Alaska Peninsula 0 120 1,200 120 –250 –200 –150 –100 –250 –200 –150 –100 km km c d –1 Vp: dry mantle km s Vp: fully hydrated forearc mantle 9 20 20 7.2 40 8 40 7.4 8.2 7.6 7.0 8.0 60 60 6.8 8.2 7 8.2 Depth (km) 80 8.0 80 7.8 8.0 100 6 100 8.0 2 8.4 8.4 8. 120 120 8.2 5 –250 –200 –150 –100 –250 –200 –150 –100 km km e f Alaska Peninsula North Cascadia 50 50 100 100 Depth (km) Sediments 150 150 Volcanics–dikes Gabbro Peridotite 0 2 4 6 8 10 0 2 4 6 8 10 –1 –1 –1 –1 H2O loss (Tg Myrs m ) H2O loss (Tg Myrs m ) Figure 2 | Predicted hydration potential and seismic velocities for individual arcs. a–e, Alaska Peninsula example (arc 1 on Supplementary Table 1). a, Predicted temperatures (from ref. 4). Yellow line, modelled slab surface; white line, upper-plate Moho from compilation. b, Maximum water capacity in 7 3 the mantle wedge predicted for a fully hydrated DMM peridotite, expressed as mass of H2O per m of mantle. c, Predicted Vp for a dry mantle (assuming anhydrous DMM composition), mineralogy estimated as described in text, and elastic moduli following ref. 22. Vp for subducting crust has been similarly 6 derived for hydrated compositions . d, Predicted isotropic P-wave velocity (Vp) for a fully hydrated forearc mantle wedge (assuming water-saturated DMM composition) corresponding to hydration in b. Contour intervals are 0.1 km s–1 for c and d; lowest velocities in d are 6.6–6.7 km s–1. e, Metamorphic dehydration of down-going slab versus slab surface depth for model D804,6, for major layers within down-going slab. The yellow field shows region below mantle forearc where vertical H2O migration would hydrate it. f, Same as e but for North Cascadia, a hot slab with substantial dehydration beneath the mantle forearc. In all calculations the pressure is assumed to be lithostatic. Sediment compaction occurs at depths less than 4–10 km below follows refs 4,13. The upper-plate Moho geometry is estimated the sea floor and drives fluids toward the trench. These depths are from a large number of seismological studies worldwide that use generally shallower than the mantle wedge10,11 except possibly in the wide-angle marine seismic imaging, receiver functions, local earth- Marianas where the upper plate is exceptionally thin and serpentine quake tomography and gravity models (detailed in Supplementary mud volcanoes occur12, perhaps tapping compaction-driven fluid. Table 1 and the accompanying Supplementary Discussion). At The H2O storage capacity of the cold mantle wedge depends on many forearcs the upper-plate Moho dips away from the trench and its volume, which in turn depends critically on the slab geometry deepens toward the volcanic arc. We have included such a sloping and the depth of the upper-plate Moho. The geometry of the slab Moho where these observations are available. 2 NATURE GEOSCIENCE | ADVANCE ONLINE PUBLICATION | www.nature.com/naturegeoscience ©2017 Mac millan Publishers Li mited, part of Spri nger Nature. All ri ghts reserved. ©2017 Mac millan Publishers Li mited, part of Spri nger Nature. All ri ghts reserved. NATURE GEOSCIENCE DOI: 10.1038/NGEO2922 PERSPECTIVE % in 8.4 02 50 Myrs 52 55 51 100 37 49 01 48 53 54 03 8.2 0 % 56 50 22 46 47 04 44 43 80 45 41 42 07 19 8 17 48 39 05 06 20 23 38 40 60 20 % 08 09 ) 33 24 27 2 31 33 –1 7.8 54 36 25 30 32 10 11 2628 29 46 42 35 12 40 7 1 43 49 40 34 14 13 (km s 40 % 36 16 15 p 7.6 37 20 V 45 17 18 0 7.4 60 % 21 Wedge Wedge 3 7.2 ? 80 % Figure 3 | Predicted per cent hydration of mantle wedges worldwide 8 produced over last 50 Myrs.
Details
-
File Typepdf
-
Upload Time-
-
Content LanguagesEnglish
-
Upload UserAnonymous/Not logged-in
-
File Pages5 Page
-
File Size-