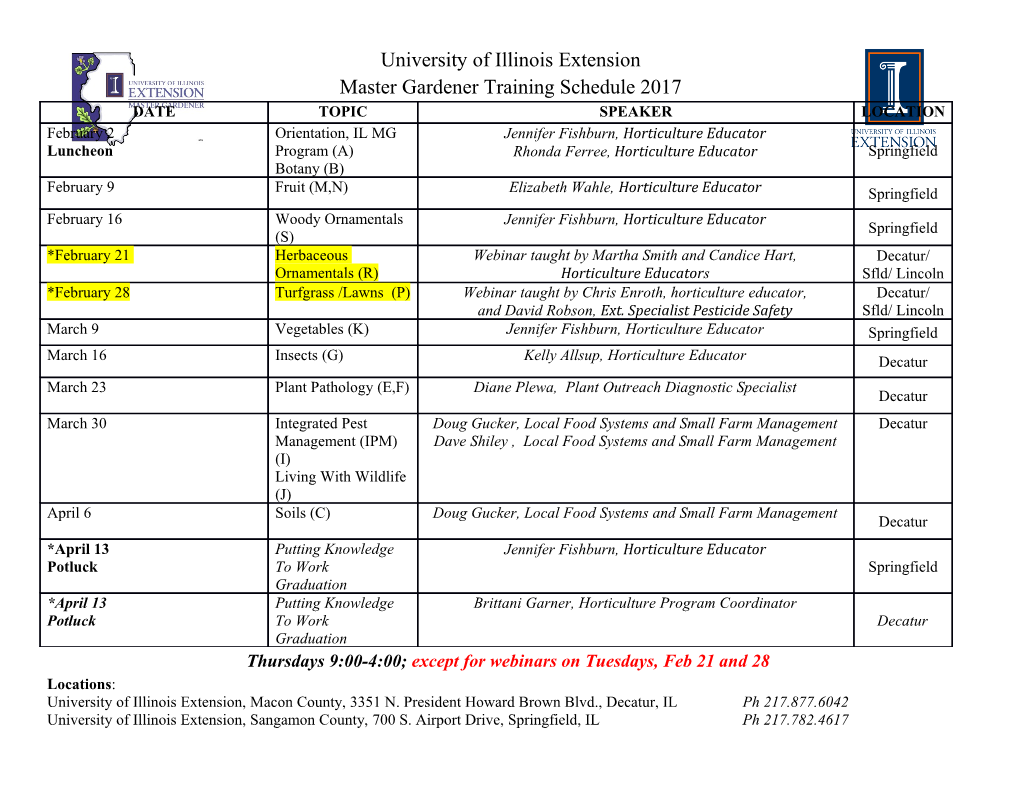
Earth and Planetary Science Letters 515 (2019) 283–290 Contents lists available at ScienceDirect Earth and Planetary Science Letters www.elsevier.com/locate/epsl Calving flux estimation from tsunami waves ∗ Masahiro Minowa a,b, , Evgeny A. Podolskiy c,d, Guillaume Jouvet e, Yvo Weidmann e, Daiki Sakakibara b,c, Shun Tsutaki f, Riccardo Genco g, Shin Sugiyama b a Instituto de Ciencias Físicas y Matemáticas, Universidad Austral de Chile, Valdivia, Chile b Institute of Low Temperature Science, Hokkaido University, Sapporo, Japan c Arctic Research Center, Hokkaido University, Sapporo, Japan d Global Institution for Collaborative Research and Education, Hokkaido University, Sapporo, Japan e Laboratory of Hydraulics, Hydrology and Glaciology, ETH Zürich, Zürich, Switzerland f Atmosphere and Ocean Research Institute, The University of Tokyo, Kashiwa, Japan g Dipartimento di Scienze della Terra, Università di Firenze, Florence, Italy a r t i c l e i n f o a b s t r a c t Article history: Measuring glacier calving magnitude, frequency and location in high temporal resolution is necessary Received 13 November 2018 to understand mass loss mechanisms of ocean-terminating glaciers. We utilized calving-generated Received in revised form 14 February 2019 tsunami signals recorded with a pressure sensor for estimating the calving flux of Bowdoin Glacier Accepted 14 March 2019 in northwestern Greenland. We find a relationship between calving ice volume and wave amplitude. Available online 1 April 2019 This relationship was used to compute calving flux variation. The calving flux showed large spatial and Editor: J.P. Avouac 5 3 −1 temporal fluctuations in July 2015 and in July 2016, with a mean flux of 2.3 ± 0.15 × 10 m d . Keywords: Calving flux was greater during periods of fast ice flow, high air temperature, and at low/falling tide, calving indicating the importance of increased longitudinal strain due to glacier acceleration and/or submarine glacier melting at the calving front. Long-term measurements with the method introduced here are promising Greenland for understanding the complex interplay of ice dynamics, melting and calving at glacier fronts. © 2019 The Author(s). Published by Elsevier B.V. This is an open access article under the CC BY-NC-ND license (http://creativecommons.org/licenses/by-nc-nd/4.0/). 1. Introduction coarse, and separating calving flux from submarine melting is dif- ficult. Ice discharge from outlet glaciers into the ocean accounts for In recent years, however, seismic measurements have been nearly half of the ice mass loss from the Greenland ice sheet (En- used to estimate calving flux and monitor the individual calving derlin et al., 2014). These glaciers have substantially retreated and events of ocean-terminating glaciers in Alaska (Bartholomaus et al., accelerated (e.g., Moon et al., 2012) under the influences of atmo- 2015), Svalbard (Köhler et al., 2016), and Greenland (Sergeant et spheric and oceanic warming (Holland et al., 2008). At the glacier al., 2016). For example, Bartholomaus et al. (2015)observed sea- front, ice mass loss occurs through frontal ablation composed of sonal to semi-diurnal variations in calving flux at Yahtse Glacier, two different physical processes: (i) calving due to mechanical Alaska by comparing passive seismic records with a calving inven- fracture of ice, and (ii) submarine melting controlled by thermody- tory, requiring tedious observation work. On a semi-diurnal time namic processes between ice and the ocean. Mass loss through the scale, tidal stresses at the calving front can influence calving rate, calving front, Q f, is commonly estimated from satellite image anal- whereas submarine melt rate modulates the calving rate on sea- ysis (e.g., Nuth et al., 2012; McNabb et al., 2015) with the equation sonal time scales at the glacier (Bartholomaus et al., 2015). Köh- below: ler et al. (2016)used a fifteen-year record of seismic signals to dL Q i − Q f estimate frontal ablation by comparing properties in seismic sig- = (1) nals with satellite-derived frontal ablation. They observed that a dt Af short-lived increase in frontal ablation was related to summer rain- where dL/dt is changes in glacier terminus position, Q is the i fall events, and seasonal peaks decayed within 1-2 months from terminus ice flux, and A is the cross sectional area of the termi- f the melt season maximum. However, mechanisms controlling the nus. However, temporal resolution using this method is relatively magnitude and the frequency of calving events are still under- investigated. Observations of glacier calving in high temporal and * Corresponding author. high spatial resolution are needed to understand the glacier mass E-mail address: [email protected] (M. Minowa). loss process from the glacier front. https://doi.org/10.1016/j.epsl.2019.03.023 0012-821X/© 2019 The Author(s). Published by Elsevier B.V. This is an open access article under the CC BY-NC-ND license (http://creativecommons.org/licenses/by-nc-nd/4.0/). 284 M. Minowa et al. / Earth and Planetary Science Letters 515 (2019) 283–290 Fig. 1. (a) Satellite image of Bowdoin Glacier in northwest Greenland, with instrument locations, and bathymetry in front of the glacier with contour intervals of 25 m. The background image was taken by Landsat 8 on 30 July 2016. (b) An example of ortho-image near the ice front obtained from UAV photogrammetry on 18 July, 2016. Blue triangles and orange circles indicate calving locations in July 2015 and July 2016 recorded by the time-lapse cameras during the field campaign. Black horizontal bar highlights the high-shear zone (Fig. S6). Calving flux histogram estimated from surface waves as a function of source-to-sensor distance with 200 m bins determined by Equation (3)in (c) July 2015 and in (d) July 2016. Gray shades highlight the location of subglacial discharge plumes which represent a mixture of subglacial freshwater and deep fjord water (Kanna et al., 2018). Similar to seismic signals, surface-water waves have a great po- tem (GPS) derived ice speed and tide phases. This study proposes tential for monitoring glacier calving (e.g., MacAyeal et al., 2009; an inexpensive, but robust and accurate methodology for continu- Amundson et al., 2008, 2012). When an iceberg breaks off into the ous and long-term measurements of calving flux. water, surface-water waves move over the ocean or lake in front of calving glaciers, propagating away from the source (MacAyeal 2. Study site et al., 2009; Minowa et al., 2018). These waves are the most dis- ◦ ◦ tinctive surface-water waves in a fjord or a lake. In the case of Bowdoin Glacier (77 41 N, 68 35 W) is located 30 km north- large-scale calving events, the resulting waves can be destructive east of Qaanaaq in northwestern Greenland (Fig. 1). The glacier (Lüthi and Vieli, 2016). These tsunami waves have been used to discharges ice into Bowdoin Fjord through a 3-km wide calving detect and locate calving events, and to estimate calving ice vol- front. The height of the ice cliff above the ocean surface is be- umes (Minowa et al., 2018). In this previous study, a positive linear tween 30 and 50 m (Jouvet et al., 2017) (Fig. S7). South-eastern relationship was found between the calving ice volume and maxi- part of the ice cliff is ∼20 m higher than north-western part of mum wave amplitude of surface-water wave (Minowa et al., 2018). ice cliff due to shallow elevated bed rock (Sugiyama et al., 2015). However, calving ice volumes were estimated from time-lapse im- Water depth near the calving front in Bowdoin Glacier is deeper ages, leading to large uncertainties. To find a more robust relation- than 100 m, and further deepens to ∼250 m about 500 m from ship, it is necessary to quantify calving ice volumes with higher the north-western side of ice front (Fig. 1) (Asaji et al., 2018). Tidal ◦ ◦ precision. Recently, Unmanned Aerial Vehicle (UAV) photogramme- data at Pituffik/Thule (76 33 N, 68 52 W) showed 1–3 m of diurnal try has been providing Digital Elevation Models (DEMs) in high and semi-diurnal tidal amplitudes. These amplitudes and phase are spatial (∼cm) and temporal (∼day) resolutions to study ocean- very similar with the local tide gauge records used in this study terminating glaciers (e.g. Jouvet et al., 2017, 2018). Such DEMs can (r2 = 0.98). −1 be used to quantify calving ice volumes with unprecedented accu- The annual mean ice speed was approximately 200 ma be- racy (e.g. compared to time-lapse image and human observation) fore 2000 (Sugiyama et al., 2015). Due to atmospheric/oceanic −1 (e.g. Bartholomaus et al., 2012; Minowa et al., 2018). warming, the glacier accelerated up to 500 ma later on, and In this study, we measured the magnitude of surface-water experienced a rapid retreat of 2 km between 2008 and 2013 waves generated by calving events at Bowdoin Glacier, an ocean- (Sugiyama et al., 2015). In the meantime, substantial glacier thin- − terminating glacier in northwestern Greenland (Fig. 1). Glacier ning of 4.1 ma 1 was observed. It was estimated that 60% of the calving was also monitored by time-lapse imagery and quantified thinning was controlled by glacier dynamics (Tsutaki et al., 2016). by high-resolution DEMs derived from UAV photogrammetry. Sur- Since 2013, the ice-front position has been relatively stable (Tsu- face wave properties were compared with calving ice volumes to taki et al., 2016; Sakakibara and Sugiyama, 2018). infer calving flux during July 2015 and July 2016. To further in- Bowdoin glacier dynamics on a daily-to-weekly scale resolution vestigate the mechanisms controlling calving, we compared the was documented by GPS, seismic measurements and UAV pho- calculated calving flux with air temperature, global positioning sys- togrammetry (Sugiyama et al., 2015; Podolskiy et al., 2016; Jouvet M.
Details
-
File Typepdf
-
Upload Time-
-
Content LanguagesEnglish
-
Upload UserAnonymous/Not logged-in
-
File Pages8 Page
-
File Size-