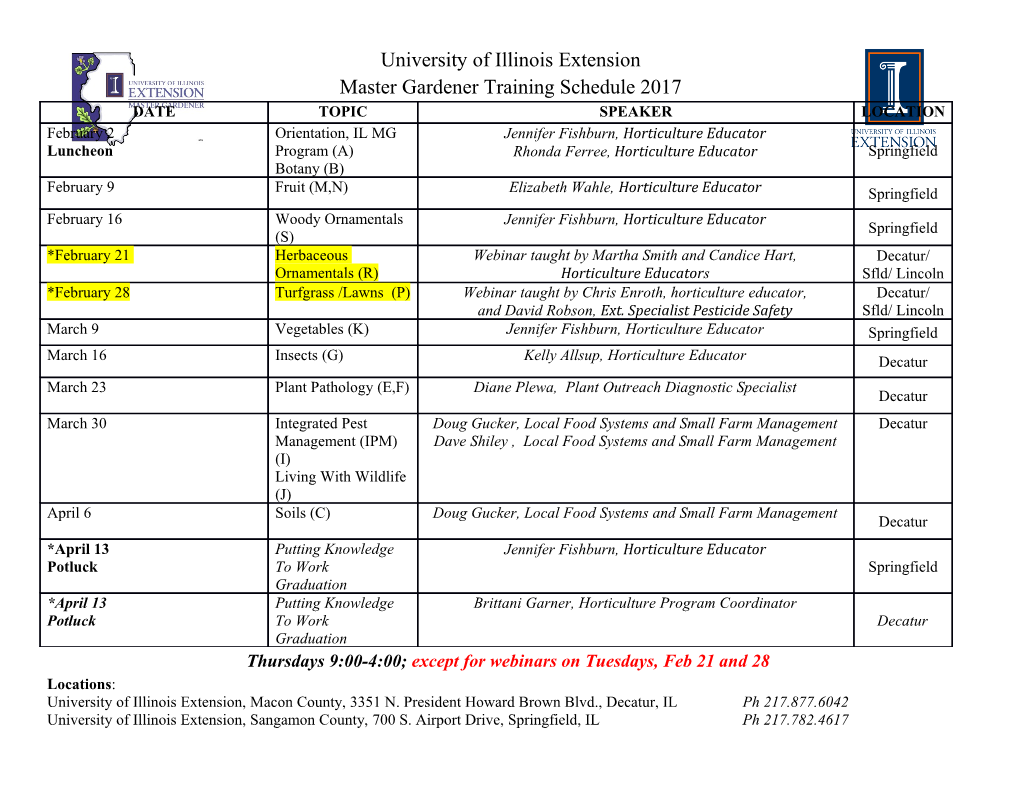
The Pennsylvania State University The Graduate School Eberly College of Science A SYNERGISTIC APPROACH TO INTERPRETING PLANETARY ATMOSPHERES A Dissertation in Astronomy and Astrophysics by Natasha E. Batalha © 2017 Natasha E. Batalha Submitted in Partial Fulfillment of the Requirements for the Degree of Doctor of Philosophy August 2017 The dissertation of Natasha E. Batalha was reviewed and approved∗ by the following: Steinn Sigurdsson Professor of Astronomy and Astrophysics Dissertation Co-Advisor, Co-Chair of Committee James Kasting Professor of Geosciences Dissertation Co-Advisor, Co-Chair of Committee Jason Wright Professor of Astronomy and Astrophysics Eric Ford Professor of Astronomy and Astrophysics Chris Forest Professor of Meteorology Avi Mandell NASA Goddard Space Flight Center, Research Scientist Special Signatory Michael Eracleous Professor of Astronomy and Astrophysics Graduate Program Chair ∗Signatures are on file in the Graduate School. ii Abstract We will soon have the technological capability to measure the atmospheric compo- sition of temperate Earth-sized planets orbiting nearby stars. Interpreting these atmospheric signals poses a new challenge to planetary science. In contrast to jovian-like atmospheres, whose bulk compositions consist of hydrogen and helium, terrestrial planet atmospheres are likely comprised of high mean molecular weight secondary atmospheres, which have gone through a high degree of evolution. For example, present-day Mars has a frozen surface with a thin tenuous atmosphere, but 4 billion years ago it may have been warmed by a thick greenhouse atmosphere. Several processes contribute to a planet’s atmospheric evolution: stellar evolution, geological processes, atmospheric escape, biology, etc. Each of these individual processes affects the planetary system as a whole and therefore they all must be considered in the modeling of terrestrial planets. In order to demonstrate the intricacies in modeling terrestrial planets, I use early Mars as a case study. I leverage a combination of one-dimensional climate, photochemical and energy balance models in order to create one self-consistent model that closely matches currently available climate data. One-dimensional models can address several processes: the influence of greenhouse gases on heating, the effect of the planet’s geological processes (i.e. volcanoes and the carbonate- silicate cycle) on the atmosphere, the effect of rainfall on atmospheric composition and the stellar irradiance. After demonstrating the number of assumptions required to build a model, I look towards what exactly we can learn from remote observations of temperate Earths and Super Earths. However, unlike in-situ observations from our own solar system, remote sensing techniques need to be developed and understood in order to accurately characterize exo-atmospheres. I describe the models used to create synthetic transit transmis- sion observations, which includes models of transit spectroscopy and instrumental noise. Using these, I lay the framework for an information content-based approach to optimize our observations and maximize the retrievable information from exo- atmospheres. First I test the method on observing strategies of the well-studied, iii low-mean-molecular weight atmospheres of warm-Neptunes and hot Jupiters. Upon verifying the methodology, I finally address optimal observing strategies for tem- perate, high-mean-molecular weight atmospheres (Earths/super-Earths). iv Table of Contents List of Figures ix List of Tables xviii Acknowledgments xix Chapter 1 Understanding and characterizing planetary atmospheres and climates 1 1.1 Remote Observations of Exoplanet Atmospheres . 2 1.1.1 Primary Transit Spectroscopy . 2 1.1.2 Secondary Transit Spectroscopy . 5 1.1.3 Overview of Exoplanet Observations . 5 1.1.4 Looking Forward: The James Webb Space Telescope . 6 1.2 Constraining Exoplanet Atmospheres Through Retrievals . 8 1.2.1 Bayesian Framework in the Context of Atmospheres . 8 1.2.2 Overview of Retrieval Techniques . 10 1.2.3 Challenges to Constraining Atmospheres of Earths and super- Earths . 12 1.3 Forward Modeling of Planetary Climates and Atmospheric Compo- sitions . 15 Chapter 2 Modeling the Planet System 17 2.1 One-Dimensional Climate Modeling . 18 2.1.1 Calculating Radiative Fluxes . 19 2.1.2 Treatment of Water Vapor and Clouds . 20 2.2 One-Dimensional Photochemical Modeling . 21 2.2.1 Model Description . 21 2.2.2 Boundary Conditions and Volcanic Outgassing . 22 v 2.2.3 The Atmospheric and Global Redox Budgets . 23 2.3 Time-dependent Evolution of Atmospheres . 27 2.3.1 Energy-Balance Climate Model Description . 27 2.3.2 Basic model equations . 27 2.3.3 Hydrogen escape . 28 Chapter 3 Early Mars: An Application to Modeling the Planet System 30 3.1 Testing the 1D H2-CO2 Greenhouse Hypothesis . 30 3.1.1 Possible Sources of Hydrogen on Early Mars . 33 3.1.1.1 Volcanic Outgassing . 33 3.1.1.2 Serpentinization . 38 3.1.1.3 Photochemical Fe Oxidation . 40 3.1.2 Early Mars Model Setup . 40 3.1.3 Photochemical Results . 42 3.1.4 Potential Warming from Other Atmospheric Constituents . 49 3.1.4.1 CO & CH4 ...................... 49 3.1.4.2 SO2 .......................... 50 3.1.5 Synergies with Current Observations . 51 3.1.5.1 S-MIF Signal Implications . 51 3.1.5.2 D/H ratios, hydrogen escape rates, and initial water inventories . 52 3.1.5.3 Tests for higher H2 outgassing rates . 54 3.1.5.4 Analyses of ancient Martian mantle redox state . 55 3.1.5.5 Analyses of Fe-oxide rich sedimentary rocks . 56 3.1.6 Conclusion . 57 3.2 Climate Cycling Caused by the Carbonate-Silicate Cycle . 59 3.2.1 Why climate limit cycles should occur on early Mars but not Earth . 59 3.2.1.1 1-D climate model calculations for present Earth . 60 3.2.1.2 The Carbonate-Silicate Cycle . 60 3.2.1.3 1-D climate model calculations for early Mars . 64 3.2.2 Energy Balance Model Setup . 64 3.2.3 Energy Balance Model Results . 65 3.2.4 Sensitivity of Models to Input Parameters . 67 3.2.4.1 Sensitivity to outgassing rates and ice albedo . 67 3.2.4.2 Sensitivity to obliquity . 68 3.2.5 Conclusion . 68 vi Chapter 4 The Planet System Observed as an Exoplanet 69 4.1 Modeling Transit Transmission Spectra . 69 4.2 Instrumental Noise Models . 71 4.2.1 Pandeia: Simulating Noise Sources . 71 4.2.2 PandExo: Simulating JWST Observations . 74 4.2.3 Benchmarking PandExo Performance . 79 4.2.3.1 NIRCam . 79 4.2.3.2 NIRISS . 80 4.2.3.3 NIRSpec . 83 4.2.3.4 MIRI . 83 4.2.4 PandExo: Simulating HST Observations . 85 4.2.5 Conclusion . 88 Chapter 5 Retrieving Information from the Spectra of the Planet System 89 5.1 The Information Content of Transit Spectra . 89 5.1.1 The Theory of IC Analysis . 91 5.1.2 Transit Transmission Spectra Models & their Jacobians . 93 5.2 Optimizing Observation Strategies for JWST: Sub-Neptunes−Hot Jupiters . 95 5.2.1 Single Observing Mode Analysis . 97 5.2.2 Validation of Covariance Matrix Approximation Against a Full Retrieval . 99 5.2.3 Two Observing Mode Analysis . 101 5.2.4 Multiple Observing Mode Analysis . 104 5.2.5 Implications for Planning Observagtions . 106 5.2.5.1 Why Two Modes Remain Superior . 106 5.2.5.2 Wavelength Coverage Versus Precision . 107 5.2.5.3 Saturation of Information . 107 5.2.6 Conclusion . 108 5.3 Optimizing Observation Strategies for JWST: Earths & Super-Earths 110 5.3.1 Noise models & Observing Strategies . 113 5.3.2 Transit Transmission Spectra Models & their Jacobians . 117 5.3.3 Information Content Analysis . 120 5.3.4 Conclusion . 121 Chapter 6 Degeneracies in Transmission Spectroscopy 124 6.1 Theoretical Setup to Investigate Degeneracies . 126 vii 6.2 A Comparison of Forward Models . 127 6.3 Implications on Future Observations of Small Planets . 132 Chapter 7 Conclusions and Future Work 135 7.1 Conclusions . 135 7.2 Future Work . 137 7.2.1 Connecting Forward Models to Retrievals . 137 7.2.2 Preparation for Direct Imaging . 139 Appendix A Photochemical Reaction Rates 140 Appendix B Energy Balance Model Parametrization 146 Bibliography 156 viii List of Figures 1.1 A schematic diagram showing the method used for observing tran- siting exoplanets. 3 1.2 Schematic showing all of the JWST spectroscopy modes as com- pared to HST’s spectroscopy (WFC3) and spectrophotometry (STIS) modes. Color coding shows approximate resolving powers of each mode. 7 1.3 Figure adapted from [Benneke and Seager, 2012]. The transmission spectra of an atmosphere with three absorbers (H2O, CH4, CO2). Each individual observable within the transmission spectra is labeled. For an atmosphere with n absorbers, there are n+4 total observables. This leads to a total of n + 4 retrievable parameters. The phrase “spectrally inactive gasses” refers to gases such as N2, H2 and He that do not exhibit strong absorption features but that may be the dominant background gas. 13 2.1 Model and grid setup for the 1-D photochemical model. 23 2.2 A schematic diagram showing the method used for balancing the ocean-atmosphere system. Φout(Red) is the flux of reductants out- gassed through volcanoes, Φrain(Red/Ox) is the flux of rained-out reductants or oxidants (including surface deposition). ΦOcean(H2) is the flux of H2 back into the atmosphere required to balance the oceanic H2 budget. An excess of reductants, such as H2S, flowing into the ocean leads to an assumed upward flux of H2. 26 3.1 Temperature-pressure profile (top) and eddy diffusion profile (bot- tom) assumed for the photochemical calculations. The temperature decreases from 273K to 147K at an altitude of 67 km and then is isothermal to the top (200 km altitude). This is consistent with the 5% H2, 95% CO2 3-bar atmosphere from Ramirez et al.
Details
-
File Typepdf
-
Upload Time-
-
Content LanguagesEnglish
-
Upload UserAnonymous/Not logged-in
-
File Pages204 Page
-
File Size-