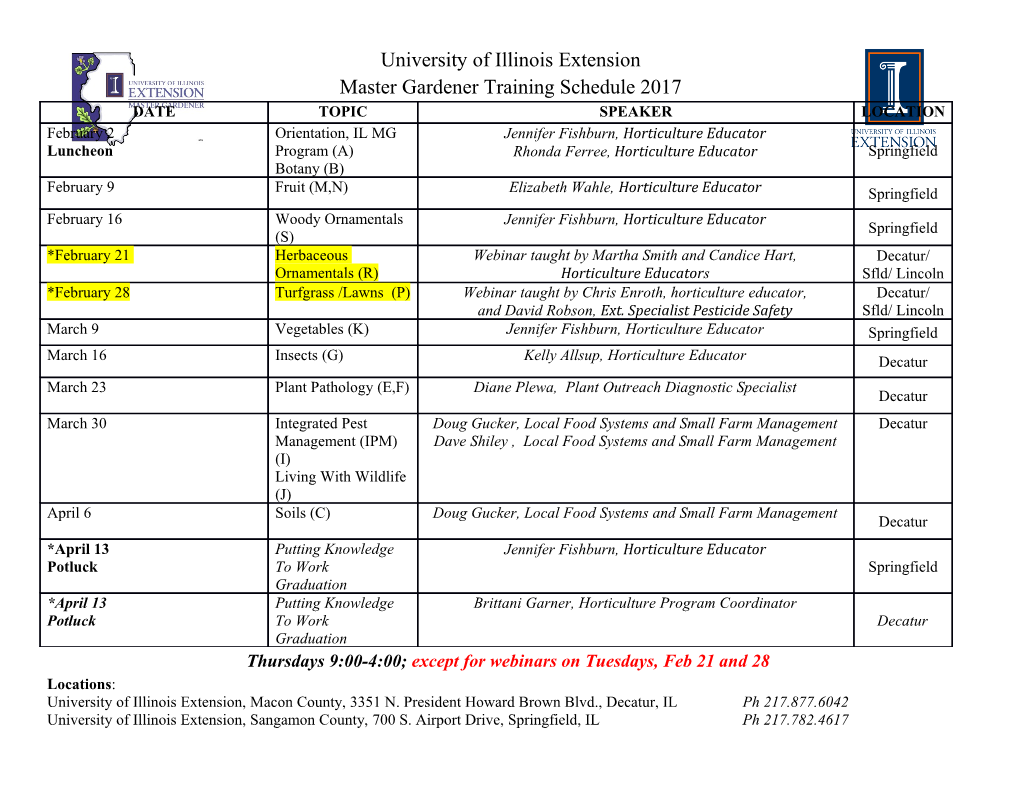
The Rheology and Morphology of Oceanic Lithosphere and Mid-Ocean Ridges R. C. Searle, J. Escartín To cite this version: R. C. Searle, J. Escartín. The Rheology and Morphology of Oceanic Lithosphere and Mid-Ocean Ridges. C.R. German; J. Lin; L.M. Parson. Mid Ocean Ridges. Hydrothermal interactions be- tween the lithosphere and the oceans, 148, American Geophysical Union, pp.63-93, 2013, Geophysical Monograph Series, 9780875904139. 10.1029/148GM03. hal-02330198 HAL Id: hal-02330198 https://hal.archives-ouvertes.fr/hal-02330198 Submitted on 23 Oct 2019 HAL is a multi-disciplinary open access L’archive ouverte pluridisciplinaire HAL, est archive for the deposit and dissemination of sci- destinée au dépôt et à la diffusion de documents entific research documents, whether they are pub- scientifiques de niveau recherche, publiés ou non, lished or not. The documents may come from émanant des établissements d’enseignement et de teaching and research institutions in France or recherche français ou étrangers, des laboratoires abroad, or from public or private research centers. publics ou privés. !"#$%"#&'&()$*+,$-&./"&'&()$&0$12#*+32$435"&6/"#.#$*+, -3,712#*+$%3,(#6 R. C. Searle Department of Earth Sciences, University of Durham, UK J. Escartín Laboratoire de Géosciences Marines (CNRS UMR7097), Institut de Physique du Globe de Paris, France The rheology of oceanic lithosphere is primarily a function of temperature, the abun- dance and distribution of lithologies and fluids, and their mechanical properties. Rheology controls the overall strength and mode of deformation. Seafloor mor- phology is the surface expression of this deformation, modified by additional processes such as volcanism. Rheological models are key to interpreting both nat- urally deformed rocks as direct indicators of deformation conditions and the result- ing morphology. Simple thermo-mechanical models have proven useful to study ridge processes, but are limited by lack of knowledge of lithospheric architecture, composition, and rheology. The mechanical properties of some components (olivine, dolerite, olivine plus melt, serpentinite) are reasonably known, but must be extended to other important materials such as alteration products and include the role of fluids and compositional variations. While the overall composition of oceanic lithosphere is relatively well known, particularly for fast-spreading ridges, the distribution and abundance of melt and alteration products is not. Though sparse, these weak phases can strongly control the overall strength, mode and localization of deformation. Thermo-mechanical models successfully reproduce observed axial relief and gen- eral faulting patterns. They provide plausible mechanisms of lithospheric behav- ior, but cannot constrain actual deformation processes. In particular, they must assume rheology, thermal structure, and composition and distribution of materials, and are non-unique. The most accurate constraints on rheology and deformation processes will come from study of naturally deformed rocks. This will guide the choice of the models used to interpret morphology and infer the detailed thermal struc- ture under ridges. INTRODUCTION Book Title The subject of the first InterRidge Theoretical Institute in Book Series Pavia, Italy, 2002, was the “Thermal regime of oceanic ridges Copyright 2004 by the American Geophysical Union and dynamics of hydrothermal circulation”. There is a fun- 10.1029/Series#LettersChapter# damental, though complex, connection between ridge mor- 1 2 CHAPTER NAME phology and the rheology and thermal regime of the litho- [Byerlee, 1968; Byerlee, 1978; Goetze, 1978]. From experi- sphere, which we review here. mental constraints on the strength of rocks at different tem- At the largest scale, the shape of the mid-ocean ridge itself perature and pressure conditions, and assuming a simple is defined by the thermal contraction of the lithosphere cre- lithology, a yield envelope for the whole lithosphere can be cal- ated at the ridge [Parsons and Sclater, 1977]. The lithosphere culated, as we discuss below [Brace and Kohlstedt, 1980; can then be deformed continuously by elastic flexure in Goetze and Evans, 1979; Kirby, 1983; Kohlstedt et al., 1995] response to applied loads such as seamounts, ocean islands, (Figure 1). These simple models provided a means to directly fracture zones, and subduction zones [Watts, 1978]. The flex- correlate rock properties with lithospheric thickness inferred ural response itself depends on the rheology of the lithosphere, from geophysical data (e.g., gravity, bathymetry, seismicity), which in turn is a function of lithospheric temperature [McNutt, but ignored the mode of deformation within the lithosphere and 1984]. At a smaller scale, the lithosphere may be deformed dis- the rheological effects of parameters such as water or litho- continuously when a fault forms [Atwater and Mudie, 1968; logical heterogeneity. Ballard and Van Andel, 1977]. The mechanics of faulting also In this approach, the mantle is modeled assuming the rhe- depends strongly on the rheology of the rock, including its ology measured for single crystals or aggregates of olivine strength and the coefficient of friction on the fault, all of crystals [Chopra, 1986; Chopra and Paterson, 1984; Durham which may be strongly dependent on temperature, pressure and Goetze, 1977; Durham et al., 1977; Evans and Goetze, and fluid content, among other parameters. Moreover, the 1979; Kohlstedt and Goetze, 1974]. For the oceanic crust, the loads resulting from faulting may cause further lithospheric rheology of diabase as determined in the laboratory [Agar flexure [Bott, 1996; Buck, 1988; Weissel and Karner, 1989]. and Marton, 1995; Caristan, 1982; Mackwell et al., 1998; The distribution, spacing, and size of faults may be controlled Shelton and Tullis, 1981] is assumed to apply to all rocks that by and provide an indication of the lithospheric thickness and compose the magmatic crust (i.e., gabbro, diabase and basalt), rheology [Shaw, 1992]. as these rocks are compositionally similar [Goetze and Evans, To fully describe and accurately model the processes occur- 1979; Kohlstedt et al., 1995]. ‘Classical’ rheological models ring at mid-ocean ridges, therefore, we need a good under- have assumed that the plastic flow law for the crust (diabase) standing and parameterization of the rheology of the lithosphere. This can be approached by a combination of lab- oratory experiments and observations on actual deformed rocks. Alternatively, we might take observations on ridge mor- phology and other parameters, and attempt to invert them to determine the underlying rheology and temperature. How- ever, given the complexities in the structures and processes involved, this link is still weak. In practice, a combined approach is necessary. In this paper, we will review current experimental and field work on the rheology of the lithosphere or its components. We will also review the morphology of mid-ocean ridges and what can be inferred about from it concerning their rheology and underlying temperature structure. 2. RHEOLOGY OF THE OCEANIC LITHOSPHERE 2.1. The Strength of the Lithosphere Figure 1. Left: variation in confining pressure (P) and temperature (T) with depth; right: corresponding yield strength envelope. The Experimental studies have revealed the physical mecha- shallow levels deform in the brittle regime, where the maximum nisms responsible for deformation in the lithosphere and pro- strength is given by Byerlee’s friction law. The brittle domain over- lies a plastic domain where deformation is accommodated by creep, vide constitutive equations to describe its mechanical behavior and shows a fast decrease in the yield strength with increasing tem- (see reviews by [Kirby, 1983] and [Kohlstedt et al., 1995]). perature and depth. The transition between the two regimes, where Early studies revealed that, to a first approximation, rocks strength is maximum, corresponds to the brittle-plastic transition deform in one of two ways: by brittle failure at low tempera- (BPT). The relative position of the Moho (6-km oceanic crustal tures and pressures (shallow depths), and by ductile or plas- thickness) is also indicated in the left panel. Calculations are for a tic deformation at higher temperatures and/or pressures, e.g., strain rate of ~10-15 s-1. AUTHOR’S NAME 3 is much weaker than that of the mantle (olivine), resulting in a decoupling weak lower crust under the ridge axis (Figure 2) [Chen and Morgan, 1990a; Shaw and Lin, 1996]. More recent experimental work shows that these models need to be revised, as the strength of dry crust may be similar to that of the man- tle (Figure 3) [Hirth and Kohlstedt, in press; Mackwell et al., 1998]. Being more complex, the continental crust is commonly modeled assuming a layered structure and using the rheol- ogy of quartz [Blacic and Christie, 1984; Jaoul et al., 1984; Kronenberg and Tullis, 1984; Mainprice and Paterson, 1984], calcite [Fredrich et al., 1989], and granite [Tullis and Yund, 1977]. More recently, experimental work has been extended to other rocks found in the lithosphere, such as serpentinite and serpentinized peridotite [Escartin et al., 2001; Escartin et al., 1997b; Reinen et al., 1994], micas [Mares and Kronenberg, 1993; Shea and Kronenberg, 1992; Shea and Kronenberg, 1993], and feldspar [Rybacki and Dresen, 2000; Tullis and Yund, 1991], among other lithologies. In addition, numerous studies demonstrate that the presence of water [Hirth and Kohlstedt, 1996; Karato et al.,
Details
-
File Typepdf
-
Upload Time-
-
Content LanguagesEnglish
-
Upload UserAnonymous/Not logged-in
-
File Pages33 Page
-
File Size-