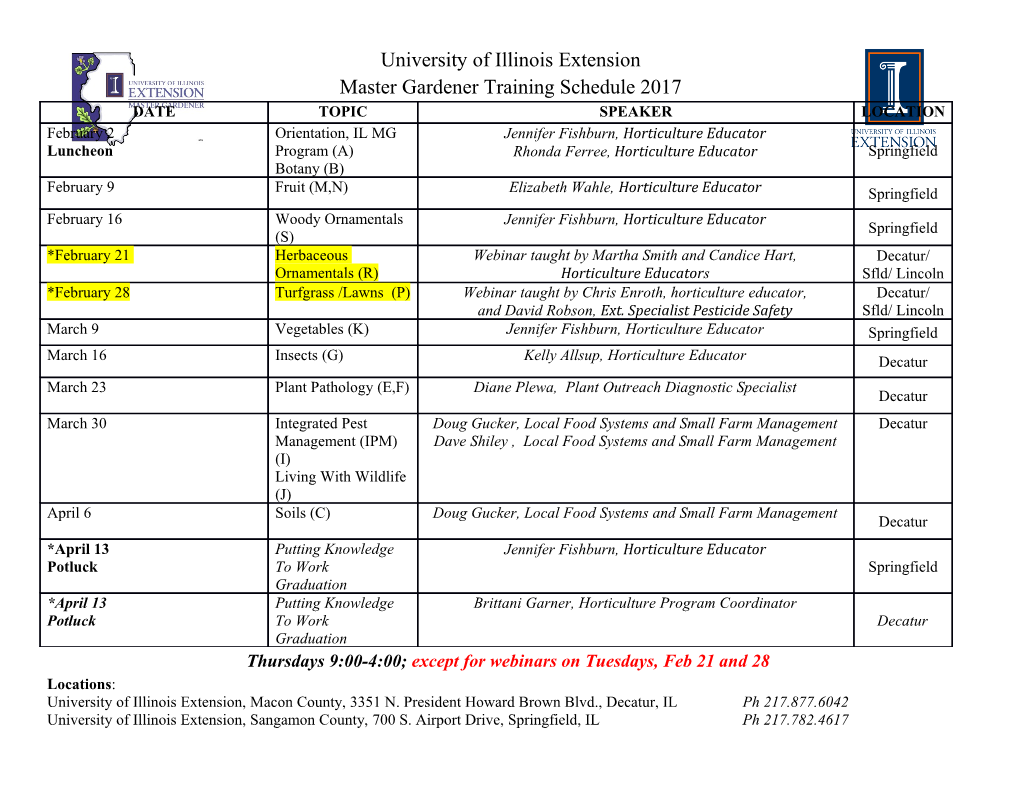
Mantle Thermal Evolution of Tidally-locked Super-Earths by Sarah E. Gelman Submitted to the Department of Earth, Atmospheric, and Planetary Sciences in Partial Fulfillment of the Requirements for the Degree of Bachelor of Science in Earth, Atmospheric, and Planetary Sciences at the Massachusetts Institute of Technology May 8, 2009 f4e 'L009 Copyright 2009 Sarah E. Gelman. All rights reserved. The author hereby grants to M.I.T. permission to reproduce and distribute publicly paper and electronic copies of this thesis and to grant others the right to do so. Signature redacted Author Department of Earth, Atmospheric, and Planetary Sciences May 8, 2009 Certified by Signature redacted Linda T. Elkins-Tanton Signature redacted Thesis Supervisor Accepted by Samuel - .,'.- Bowring 2' Chair, Committee on Undergraduate Program MASSACHUSESINSTITUTE OF TECHNOLOGY OCT 2 4 2017 The author hereby grants to MWT permission to reproduce and to distuibute publicly paper and electronic copies of this thesis document ip LIBRARIES whole or in part in any medium now known or hereafter created. ARCHIV. ABSTRACT Mantle Thermal Evolution of Tidally-locked Super-Earths by Sarah E. Gelman Advisors: Linda T. Elkins-Tanton, Sara Seager Most super-Earth (mass < 10MD) detection techniques are biased towards massive planets with close-in orbits. A planet's orbital decay timescale decreases with a lower semi-major axis, thereby providing a high probability of detecting exoplanets which are in tidal-lock with their star. We model the effect of fixed stellar flux on an Earth- like planet's mantle convection structure and evolution using an axisymmetric finite element fluid convection code, SSAXC. Three punctuating evolutionary steps have been identified. First, a sequence of three initial downwellings form at the antistellar point, the substellar point, and at the terminator. After approximately 250,000 years, lithospheric instabilities drip down into the mantle, inducing pervasive small scale convective cells. Finally, after 3.5 billion years of planetary evolution, a cold region (~ 300'C) develops at the antistellar point, spanning the depth of the mantle, and inducing a pseudo-Mode 1 convective pattern. Though initial models have focused on an incoming stellar flux equivalent to that at the Earth, we also discuss the possibility of steady-state partial magma oceans in the substellar region induced by higher stellar fluxes. We propose that, though these tidally-locked planets may not be considered habitable in general, they may contain locally habitable regions due to their variety of long-lived extreme environments. Contents Table of Contents 3 List of Figures 4 List of Tables 5 1 Introduction to Super-Earths 6 1.1 Detection Techniques for Extrasolar Planets ........... ....... 6 1.2 Survey of Earth-Sized Exoplanet Discoveries .................. 10 1.3 Survey of Super-Earth Models .......................... 11 1.4 The Significance of Synchronous Rotation ............ ....... 12 2 Numerical Methods 15 2.1 Introduction to SSAXC ... ......... ......... ......... 15 2.2 Derivation of Boundary Conditions ......... ......... ..... 16 2.3 Input Parameters and Simplifications .. ......... ......... .. 18 3 Results 20 3.1 Conduction and Radiation Only .......... .............. 20 3.2 Implementation of Convection ... ......... ......... ..... 21 3.3 Mode 1 Convection and Long-Term Evolution ............. .... 28 4 Discussion: Partial Magma Oceans 31 5 Conclusions 35 Bibliography 37 A SSAXC Modifications 42 B Magma Pond Stability Code 50 3 List of Figures 1.1 Illustration of Transit Light Curve Method ... ................ 7 1.2 HD 209458 Light Curve ........................ ..... 8 1.3 51 Pegasi b Radial Velocity ..... ...................... 9 1.4 OGLE-2005-BLG-390 Gravitational Microlensing ............... 10 1.5 Synchronous Rotation Illustration .... ............. ....... 13 2.1 SSAXC Grid Setup ... ......... ......... ......... .. 16 3.1 Initial Conductive Results ........ ......... ......... .. 21 3.2 4-D Early Convective Results ... ......... ......... ..... 22 3.3 Highlight of Initial Convective Downwellings .......... ........ 23 3.4 Downwellings and Small Scale Convection ............ ....... 24 3.5 Hemispheric Dichotomy from Lithospheric Drips ......... ....... 26 3.6 Stability of Surface Liquid Water ...... ......... ......... 27 3.7 4-D Long-term Convective Results ............ ........... 29 4.1 Magma Pond Stability Timescales .... ......... ......... .. 33 5.1 Image of Gliese 581 ..... ......... ......... ......... 36 4 List of Tables 1.1 Detected Super-Earths . .. ..................... .11 2.1 SSAXC Input Parameters ............ ................ 19 4.1 Magma Pond Stability Parameters .............. ......... 32 5 1 Introduction to Super-Earths To date, 347 extrasolar planets have been discovered, most of them large, Jupiter-mass gaseous bodies orbiting close to their star. Although the detection of these planets is in- triguing and challenges traditional views on planetary evolution and solar system formation, it is the by-product of a larger search for more Earth-like, rocky worlds beyond our solar system. Detection techniques preferentially discover these larger gas giant planets; however, as these techniques are continually refined, the minimum limit of detectability is closing in on Earth-mass planets. Indeed, only since 2005 have we been able to detect planets which may be small enough to be considered predominantly rocky (Rivera et al., 2005; Valencia et al., 2007a). Since then, the number of identified potentially rocky planets is growing, and with new space satellite missions underway (for example, Kepler, launched March 6, 2009), this number can be expected to continue its upward trend. 1.1 Detection Techniques for Extrasolar Planets There will be three main ways to detect Earth-mass exoplanets in the near future: (1) transit light curve analysis, (2) radial velocity variations, and (3) gravitational microlensing events (e.g., Griest and Safizadeh, 1998; Woolf and Angel, 1998; Marcy and Butler, 1998; Santos, 2008). These techniques provide complementary information, and each has its own sensitivities and limitations. In the transit light curve method, the photon flux of a star is observed and plotted against time. A planet is detected when the intensity of the star drops off as an orbiting planet passes directly between the star and the observer on Earth (Fig. 1.1). This orbital transit will not be observed for every planet because of the random orientation of stellar 6 7 Figure 1.1 Schematic explanation of an exoplanet transit light curve. From the point of view of an observer on Earth, the exoplanet (shown in blue) transits in front of the star (shown in yellow) as it orbits. In this particular cartoon, the planet is moving from left to right, but in reality stellar disk orientations are random and so this is not necessarily the case for any given exoplanet. The corresponding fitted light curve data of the transit is shown just below. The black line represents the flux of photons an observer records as the transit proceeds. The overall intensity from the star drops as the planet transits, and is restored afterwards. Figure simplified from Brown et al. (2001). disks. This technique will thus favor planets whose probability of a transit observable from Earth is high: planets close to their star, very large in radius, and low in albedo. Since the transit light curve method is dependent on the absolute intensity of light from a star, atmospheric effects can severely distort a ground-based telescope's data quality. Space-based observations are not necessary to detect a planet, but do provide a level of precision which greatly increases our ability to constrain planetary and orbital characteristics, especially with smaller-sized objects. Of the 347 exoplanets, 59 were detected with the transit light curve method. An example of a light curve obtained by Brown et al. (2001) using the Hubble Space Telescope is shown in Fig. 1.2. Measuring the variability in the radial velocity of a star provides a higher level of precision than the transit light curve method in detecting extrasolar planets. In any planetary system, 8 1.000 0.995- 0 0.990- 0.985 -0.10 -0.05 0.00 0.05 0.10 time from center of transit (days) Figure 1.2 Example of transit light curve data obtained by Brown et al. (2001) of a large (Mp = 1.347 Rj) gaseous extrasolar planet orbiting HD 209458. Orbital period is 3.52474 days. Data was obtained from the Hubble Space Telescope over four transit events. both star and planet are orbiting a common center of mass. Thus, as a planet makes a complete revolution a slight wobble movement can be observed via a Doppler shift in the stellar spectra, assuming the orbital plane is oriented optimally. When a star moves towards the Earth in its orbit, we measure an increase in the frequency of the star's photons, or, in other words, a slight blueshift. When the star moves away from the Earth, we observe a redshift. Even though the radial velocity method is still biased towards the detection of large, close-in planets (since more massive bodies have greater gravitational potential), these measurements have been the most successful. So far 321 planets have been detected by radial velocity perturbations. An example, 51 Pegasi b (the first exoplanet ever detected), is shown in Fig. 1.3. A third important detection technique is the analysis of gravitational microlensing events. In this case, due to differing relative velocities of objects in the Milky Way,
Details
-
File Typepdf
-
Upload Time-
-
Content LanguagesEnglish
-
Upload UserAnonymous/Not logged-in
-
File Pages52 Page
-
File Size-