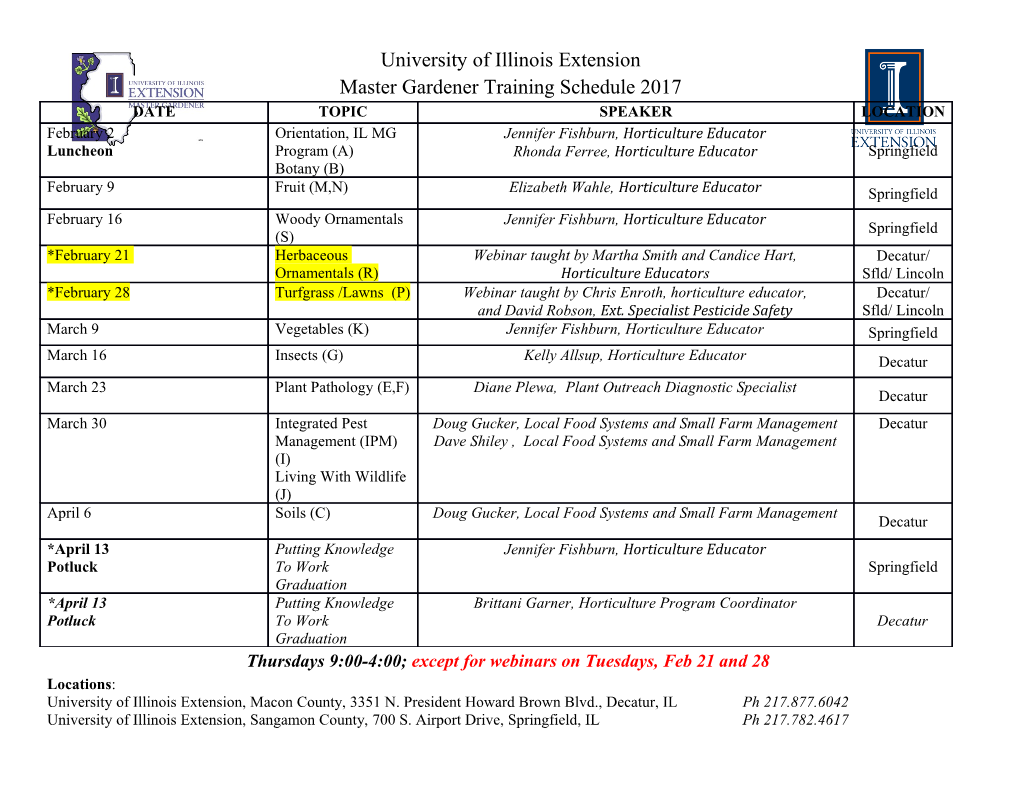
Engineered Living Materials: Engineered Living Materials: Prospects and Challenges for Using Biological Systems to Direct the Assembly of Smart Materials The Harvard community has made this article openly available. Please share how this access benefits you. Your story matters Citation Nguyen, Peter Q., Noémie#Manuelle Dorval Courchesne, Anna Duraj#Thatte, Pichet Praveschotinunt, and Neel S. Joshi. 2018. Engineered Living Materials: Engineered Living Materials: Prospects and Challenges for Using Biological Systems to Direct the Assembly of Smart Materials. Advanced Materials 30, no. 19. Citable link http://nrs.harvard.edu/urn-3:HUL.InstRepos:40992633 Terms of Use This article was downloaded from Harvard University’s DASH repository, and is made available under the terms and conditions applicable to Open Access Policy Articles, as set forth at http:// nrs.harvard.edu/urn-3:HUL.InstRepos:dash.current.terms-of- use#OAP Revised Manuscript Part1 1 2 3 4 1 5 6 7 2 8 9 3 10 11 12 4 13 14 5 15 16 6 17 18 19 7 20 21 8 22 23 24 9 Engineered Living Materials: Prospects and Challenges for Using Biological Systems to 25 26 10 Direct the Assembly of Smart Materials 27 28 29 11 30 31 12 Peter Q. Nguyen, Noémie-Manuelle Dorval Courchesne, Anna Duraj-Thatte, Pichet 32 33 34 13 Praveschotinunt, and Neel S. Joshi* 35 36 14 37 38 15 39 40 41 16 *Corresponding Author: Dr. Neel S. Joshi 42 17 School of Engineering and Applied Sciences / 43 18 Wyss Institute for Biologically Inspired Engineering 44 19 Harvard University 45 46 20 Cambridge, MA 02138, USA. 47 21 48 22 Tel: (617) 432-7732 49 23 E-Mail: [email protected] 50 51 24 52 53 25 54 55 26 Keywords : Engineered Living Materials, Synthetic Biology, Biomaterials, Smart Materials, 56 57 58 27 Self-healing. 59 60 61 62 63 64 65 1 2 3 4 28 Abstract 5 6 7 29 Vast potential exists for the development of novel, engineered platforms that manipulate biology 8 9 30 for the production of programmed advanced materials. Such systems would possess the 10 11 12 31 autonomous, adaptive, and self-healing characteristics of living organisms but would be 13 14 32 engineered with the goal of assembling bulk materials with designer physicochemical or 15 16 33 mechanical properties, across multiple length scales. Early efforts towards such Engineered Living 17 18 19 34 Materials (ELMs) are reviewed here, with an emphasis on the engineered bacterial systems, living 20 21 35 composite materials which integrate inorganic components, successful examples of large-scale 22 23 24 36 implementation, and production methods. In addition, a conceptual exploration of the fundamental 25 26 37 criteria of ELM technology and its future challenges is presented. Cradled within the rich 27 28 29 38 intersection of synthetic biology and self-assembling materials, the development of ELM 30 31 39 technologies will allow us to leverage the power of biology to grow complex structures and objects 32 33 34 40 using a palette of bionanomaterials. 35 36 41 37 38 42 Introduction 39 40 41 43 Next generation advanced materials must include ‘smart’ functional properties that surpass 42 43 44 existing capabilities, such as adaptation to environmental cues, the ability to dynamically switch 44 45 46 45 between different material states, and self-healing capabilities. Materials with many of these 47 48 46 properties have already been developed by living systems over the last ~4.5 billion years, as they 49 50 51 47 have created biological structures for the modification of their environment and survival. From a 52 53 48 materials standpoint, cells can be considered as nanomaterials factories that constantly sense their 54 55 49 environment, draw from a plethora of energy sources and simplistic molecular building blocks, 56 57 58 50 refashion these molecules into new structurally and functionally more complex materials, and 59 60 61 62 2 63 64 65 1 2 3 4 51 maintain these materials over time. Given the amazing complexity of materials that biology is 5 6 7 52 capable of producing, it is no surprise that bioinspired approaches to the design and engineering 8 9 53 of materials is a fertile area of exploration. A core strategy that living systems employ is the use 10 11 12 54 of biomolecular self-assembly to create large structures from molecular building blocks that have 13 14 55 evolved to associate in a precise manner. Nowhere is this more apparent than the amazing accuracy 15 16 56 of the self-assembling material employed by living systems for long-term information storage – 17 18 19 57 DNA. Thus, robust bottom-up fabrication methods are a fundamental aspect of biological 20 21 58 materials. Much effort in the past few decades has focused on unravelling the mechanisms and 22 23 24 59 regulation of biological self-assembly systems. As we have gleaned these core principles, they 25 26 60 have been successfully applied in engineering efforts, creating artificial self-assembling materials 27 28 29 61 composed of peptides, proteins, DNA, and carbohydrates. However, many of these engineered 30 31 62 materials utilize biomolecular building blocks under highly controlled conditions – they are often 32 33 34 63 purified and assembled in vitro. Unfortunately, many of the unique properties of living systems 35 36 64 are lost under such conditions. A parallel engineering effort that has occurred in the past decade is 37 38 65 Synthetic Biology, which focuses on applying knowledge of genetic regulation and biological 39 40 41 66 systems towards the systematic development of forward-engineered artificial biological circuits. 42 43 67 44 45 46 68 A rich area has blossomed at the interface of self-assembling biomaterials and synthetic biology. 47 48 69 Broadly, this area can be classified as biohybrid materials and encompasses any composite 49 50 51 70 material that has a biologically-derived component and a synthetic component. The biological 52 53 71 component may be purified biomolecules, such as proteins or DNA, or living cells. The synthetic 54 55 72 component could be organic or inorganic polymers, minerals, ceramics, or even metals. Examples 56 57 58 73 of such biohydrid materials/devices include cells that are spatially embedded or encapsulated in 59 60 61 62 3 63 64 65 1 2 3 4 74 polymeric structures, such as stimuli-responsive materials composed of cells covalently linked 5 6 [1] [2-6] 7 75 with polymers , microfluidic devices recapitulating complex organ-level systems , propulsion 8 9 76 of microparticles using attached bacteria[7], wearables harboring genetically engineered cells[8], 10 11 [9-11] [12, 13] 12 77 encapsulated cells in fibers or sol-gels , and optically positioned cells on 3D microscaffold 13 14 78 structures[14]. However, in these examples of biohybrid materials, biological component, whether 15 16 79 it is biopolymeric or includes living cells, is just one element of the engineered structure and does 17 18 19 80 not actively create or modulate the bulk structure of the material. This can be contrasted with actual 20 21 81 biological systems, in which cells not only produce the material through a combination of 22 23 24 82 organized self-replication and biopolymer synthesis, but also modify its properties through 25 26 83 context-dependent active processes over its lifetime. 27 28 29 84 30 31 85 Here, we provide a comprehensive review of an emerging area within biohybrid materials, 32 33 34 86 Engineered Living Materials (ELMs). We define ELMs as engineered materials composed of 35 36 87 living cells that form or assemble the material itself, or modulate the functional performance of 37 38 88 the material in some manner (Figure 1). A key difference between ELMs and other biohybrid 39 40 41 89 devices is that in ELMs, the living cells act as materials factories, drawing upon energy feedstocks 42 43 90 from their environment to create biopolymeric building blocks and direct the formation of, or 44 45 46 91 maintain, the desired material. The engineered aspect of an ELM may take on a variety of forms, 47 48 92 such as genetic engineering of the material components or simply spatial/mechanical engineering 49 50 51 93 to restrict or position the cells. The ELM may be composed predominantly of the cellular biomass 52 53 94 or could secrete materials that form a large part of the structure (such as in biofilms). Furthermore, 54 55 95 ELMs may be designed to integrate other inorganic polymers, particles, or scaffolds into the 56 57 58 96 material in some way as part of the material assembly process. Unlike most other self-assembled 59 60 61 62 4 63 64 65 1 2 3 4 97 biomaterials that utilize purified components, ELM should be able to robustly direct the formation 5 6 7 98 of the material in complex conditions containing a multitude of components, such as nutrient 8 9 99 feedstocks or metabolic wastes. Because they are living, ELMs may also allow for significant 10 11 12 100 programmed stimuli-responsive changes over the viable lifetime of the cellular components. If 13 14 101 desired, an ELM can, at a particular juncture, be processed to remove or kill the living cells, 15 16 102 retaining the assembled materials without concern for continued maintenance or potential 17 18 19 103 biohazard threats of the cells. 20 21 104 22 23 24 105 The bioinspiration for ELMs can be found throughout nature, in how living systems manipulate 25 26 106 and reshape the environment around them for their own gain (Figure 2).
Details
-
File Typepdf
-
Upload Time-
-
Content LanguagesEnglish
-
Upload UserAnonymous/Not logged-in
-
File Pages85 Page
-
File Size-