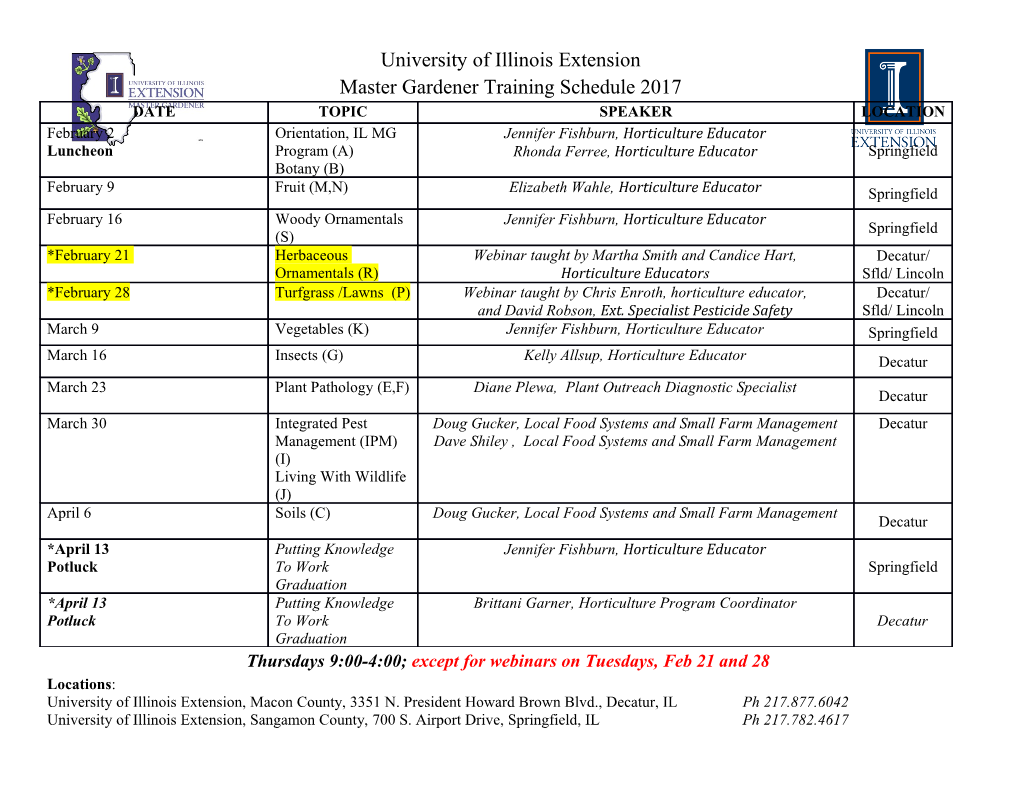
Lie algebras, their representation theory and GLn Minor Thesis Greta Panova June 20, 2008 Contents 1 Introduction 1 2 Lie Algebras 2 2.1 Definitions and main theorems . 2 2.2 Representations of Lie algebras . 7 2.3 Representations of sl(2;F ) ..................................... 9 3 Lie Groups and their Lie algebras 11 4 Representations of semisimple Lie algebras 15 4.1 Root space decomposition . 15 4.2 Roots and weights; the Weyl group . 19 5 The special linear Lie algebra sl(n; C) and the general linear group GLn(C) 22 5.1 Structure of sl(n; C) ......................................... 22 5.2 Representations of sl(n; C)...................................... 24 5.3 Weyl's construction, tensor products and some combinatorics . 25 n 5.4 Representations of GLn(C ) .................................... 27 5.5 Some explicit construction, generators of SλV ........................... 28 6 Conclusion 30 A Schur functors, Weyl's construction and representations of GLn(C) 30 1 Introduction The goal of this minor thesis is to develop the necessary theory of Lie algebras, Lie groups and their representation theory and explicitly determine the structure and representations of sln(C) and GLn(C). My interest in the representations of GL(V ) come from their strong connection to combinatorics as developed in Chapter 7 and its appendix in [3]. Even though the irreducible holomorphic representations of GL(V ) can be derived without passing through the general theory of Lie algebras, as Schur did in his dissertation, any attempt to generalize the result or to obtain analogous results for the unitary or symplectic groups will pass through the theory of Lie algebras. Here we will develop the basic theory of Lie algebras and their representations, focusing on semisimple Lie algebras. We will develop most of the necessary theory to show facts like complete irreducibility of representations of semisimple Lie algebras, develop the theory necessary to decompose the lie algebras into root spaces and use these root spaces to decompose representations into weight spaces and list the irreducible 1 representations via weights. We will establish connections between Lie groups and Lie algebras, which will, for example, enable us to derive the irreducible representations of GL(V ) through the ones for gl(V ). In our development of the basic theory of Lie algebras we will follow mostly [2], while studying Lie groups, roots and weights, sl(n; S) we will follow [1]. We will encounter some combinatorial facts which will be taken for granted and whose proofs are found in [3]. 2 Lie Algebras In this section we will follow [2]. We will develop the basic theory of Lie algebras and later we'll establish how they arise from Lie groups and essentially motivate their existence. 2.1 Definitions and main theorems We will, of course, start with the definition of a Lie algebra. Definition 1. A Lie algebra is a vector space over a field F endowed with a bracket operation L × L ! L denoted (x; y) ! [xy], which satisfies the following axioms: (L1) [xy] is bilinear, (L2) [xx] = 0 for all x 2 L, (L3) [xy] satisfies the Jacobi identity [x[yz]] + [y[zx]] + [z[xy]] = 0 for all x; y; z 2 L. A homomorphism (isomorphism) of Lie algebras will be a vector space homomorphism (resp. isomor- phism) that respects the bracket operation, a subalgebra would a subspace closed under the bracket operation. An important example of Lie algebra is the general linear algebra gl(V ), which coincides as a vector space with End V (or Mn - space of n×n matrices) and has a bracket operation defined as [XY ] = XY −YX (it is a straightforward check to verify the axioms). Next we can define the special linear algebra sl(V ) as the subspace of End V of trace 0 endomorphisms and the same bracket operation, it is clearly a subalgebra of gl(V ). Similarly we can define other subalgebras of gl(V ) by imposing conditions on the matrices, e.g. uppertriangular, strictly uppertriangular, diagonal. Another important example of a gl(U) subalgebra, where U is a vector space endowed with a bilinear operation (denoted as multiplication), is the algebra of derivations Der U. It is first of all the vector space of linear maps δ : U ! U, such that δ(XY ) = Xδ(Y ) + δ(X)Y . In order to verify that this is a subalgebra we need to check that [δδ0] 2 DerU. For the sake of exercise we will do that now. We need to check that [δδ0] = δδ0 − δ0δ satisfies the derivation condition (it is clear it's a linear map), so for X; Y 2 U we apply the derivation rule over and over 1. Now if we let U = L with the bilinear operation defined by the bracket of L, we can talk about Der L. Some of these derivations arise as endomorphism ad x : L ! L given by ad x(y) = [xy]. It is a derivation, since by the Jacobi identity ad x(yz) = [x[yz]] = −[y[zx]] − [z[xy]] = [y[xz]] + [[xy]z] = [y ad x(z)] + [ad(y)z]. We can now make a Definition 2. The map L ! Der L given by x ! ad x, where ad x(y) = [xy] for all y 2 L, is called the adjoint representation of L. Continuing with the definitions we need to define ideals of a Lie algebra. The notion is the same as ring ideals, just that multiplication is given by the bracket, in other words I is an ideal of L if for any x 2 L and y 2 I [xy] 2 I. Two important ideals are the center Z(L) = fz 2 Lj[xz] = 0 for all x 2 Lg and the derived algebra [LL] consisting of all linear combinations of [xy]. Sums and products (given by the bracket) of ideals are clearly also ideals. A Lie algebra with no nontrivial ideals (i.e. 0 and itself) and with [LL] 6= 0 is called simple, in this case [LL] 6= 0 necessarily implies [LL] = L. We define the normalizer of a subalgebra K of 1[δδ0](XY ) = δ(δ0(XY )) − δ0(δ(XY )) = δ(δ0(X)Y + Xδ0(Y )) − δ0(δ(X)Y + Xδ(Y )) = δ(δ0(X)Y ) + δ(Xδ0(Y )) − δ0(δ(X)Y ) − 0 0 0 0 0 0 0 0 0 0 δ (Xδ(Y )) = δ(δ (X))Y +δ (X)δ(Y )+δ(X)δ (Y )+Xδ(δ (Y ))−δ (δ(X))Y −δ(X)δ (Y )−δ (X)δ(Y )−Xδ (δ(Y )) = X(δδ (Y )− δ0δ(Y )) + (δδ0(X) − δ0δ(X))Y = X[δδ0](Y ) + [δδ0](X)Y 2 L as NL(K) = fx 2 Lj[xK] ⊂ Kg and the centralizer of a subset X of L as CL(X) = fx 2 Lj[xX] = 0g, both of them turn out to be subalgebras of L by the Jacobi identity 2 We need to define what we mean by a representation of a Lie algebra since this is the ultimate goal of this paper. A representation of L is a homomorphism φ : L ! gl(V ). The most important example of which is the already mentioned adjoint representation ad L :! gl(L). Since linearity is clear, all we need to check is that it preserves the bracket, which again follows from the Jacobi identity 3. We proceed now to some very important concepts in the theory of Lie algebras. We call a Lie algebra L solvable if the sequence of ideals L(0) = L; L(1) = [LL];:::;L(n) = [L(n−1)L(n−1)] eventually equals 0. Note for example that simple algebras are not solvable, as L(n) = L for all n. Here is the place to introduce the subalgebra of gl(n; F ) t(n; F ) of upper triangular n × n matrices and the subalgebra n(n; F ) of strictly upper triangular matrices. We notice that [t(n; F )t(n; F )] ⊂ n(n; F ), and that taking the commutator of two matrices A and B with entries ai;j; bi;j = 0 if j − i ≤ k for k > 0 will result in C = AB − BA with ci;j = 0 for j − i ≤ k + 1 and continuing this way we will eventually get a 0 matrix (e.g. for k > n), so these two algebras are solvable. We note here some easy facts. If L is solvable, so are all its subalgebras and homomorphic images. Also, if I is a solvable ideal of L and L=I is solvable, then so is L. This can be shown by considering the quotient map π : L ! L=I. Since (L=I)(n) = 0, then so is 0 = (π(L))(n) = π(L(n)), so L(n) ⊂ Ker π = I. Since I(k) = 0 for some k, then L(n+k) = (L(n))(k) = 0. As a consequence of this fact we have that if I and J are solvable ideals of L, then since (I + J)=J ∼ I=(I \ J) is solvable as a quotient of I, by the what we just proved I + J is also solvable. This allows us to make one of the most important definitions, that of semisimplicity. If S is a maximal (not contained in any other such) solvable ideal of L, then for any other solvable ideal I we would have S + I solvable, so by the maximality of S, S + I = S and hence I ⊂ S, showing that S must be unique. We denote S by Rad L, the radical of L and make the Definition 3. A Lie algebra L is called semisimple if its radical is 0, Rad L = 0. We now define another very important notion to play a role later.
Details
-
File Typepdf
-
Upload Time-
-
Content LanguagesEnglish
-
Upload UserAnonymous/Not logged-in
-
File Pages32 Page
-
File Size-