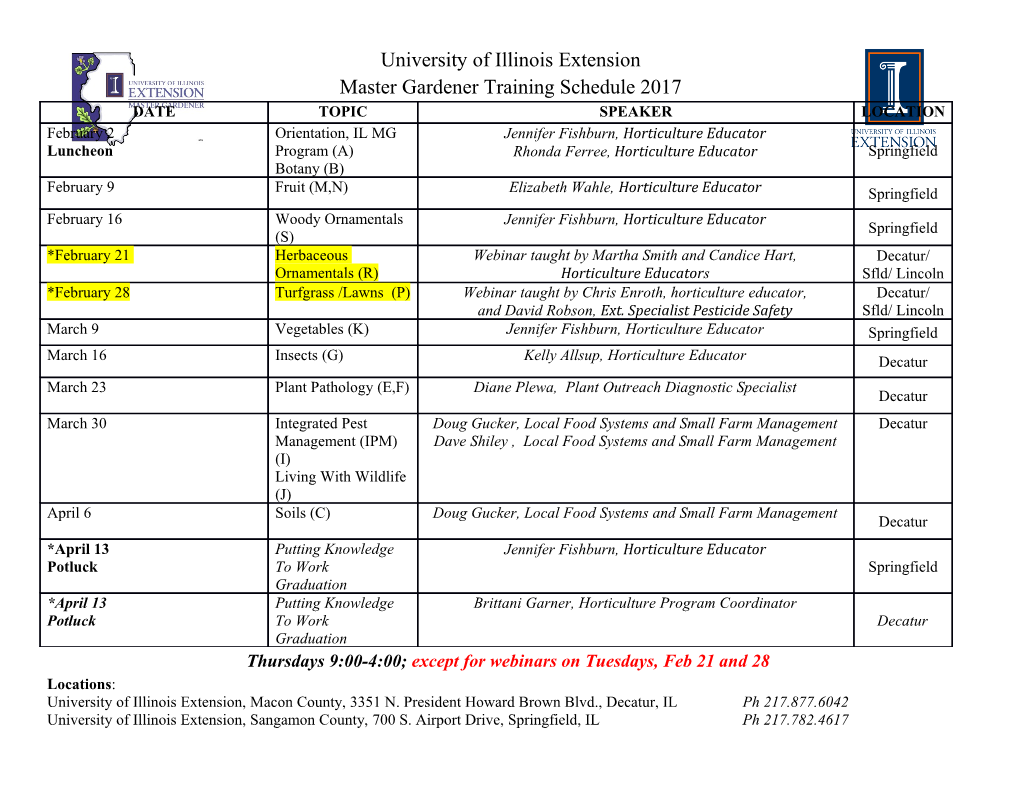
2012 Matthew Schwartz II-4: Spinor solutions and CPT 1 Introduction In the previous lecture, we characterized the irreducible representations of the Lorentz group O(1,3). We found that in addition to the obvious tensor representations φ, A µ , h µν etc., there are a whole set of spinor representations, such as Weyl spinors ψL , ψR. A Dirac spinor ψ trans- forms in the reducible 1 , 0 0, 1 representation. We also found Lorentz invariant 2 ⊕ 2 Lagrangians for spinor fields , ψ( x) . The next step towards quantizing a theory with spinors is to use these Lorentz group representations to generate irreducible unitary representations of the Poincaré group. Recall that unitary representations of the Poincaré group are induced from representations of its little group which stabilizes pµ, SO(3) or ISO(2), for the massive or massless cases respec- tively. For massive particles, we expect 2 J + 1 degrees of freedom and for massless particles, 2 degrees of freedom. In the vector case, we found that there were ambiguities in what the free Lagrangian was (it could have been aA µ A µ + bA µ∂µ∂νAν for any a and b), but we found that there was a unique Lagrangian which propagated the correct degrees of freedom. We then solved i the free equations of motion for a fixed momentum pµ generating 2 or 3 polarizations ǫ µ( p) . These solutions, which were representations of the little group, if known for every value of pµ, induce representations of the full Poincaré group. For the spin 1 case, there is a unique free Lagrangian (up to Majorana masses) which auto- 2 matically propagates the right degrees of freedom. In this sense, spin 1 is easier than spin 1 , 2 since there are no unphysical degrees of freedom. The mass term couples left- and right-handed spinors, so it is natural to use the Dirac representation. As in the spin 1 case, we will solve the i free equations of motion to find basis spinors, us ( p) and vs ( p) (analogs of ǫ µ) which we will use to define our quantum fields. As with complex scalars, we will naturally find both particles and antiparticles in the spectrum with the same mass and opposite charge: these properties fall out of the unique Lagrangian we can write down. A spinor can also be its own antiparticle, in which case we call it a Majorana spinor. As we saw, since particles and anti-particles have opposite charges Majorana spinors must be neutral. We will define the operation of charge conjugation C as taking particles to antiparticles, so Majorana spinors are invariant under C. After introducing C, it is natural to continue to discuss how the discrete symmetries parity, P, and time-reversal, T, act on spinors. 2 Chirality, helicity and spin In a relativistic theory, spin can be a confusing subject. There are actually three concepts asso- ciated with spin: spin, helicity and chirality. In this section we define and distinguish these dif- ferent quantities. Recall from the previous lecture that the Dirac equation ( iD m) ψ = 0 implies − 2 e µν 2 ( i∂µ eA µ) Fµν σ m ψ = 0 (1) − − 2 − and for the conjugate field ψ¯ = ψ † γ0 2 e µν 2 ψ¯ ( i∂ µ + eA µ) + Fµν σ m = 0 (2) 2 − Thus ψ¯ , is a particle with mass m and charge opposite to ψ; that is, ψ¯ is the antiparticle of ψ. We will often call ψ an electron and ψ¯ a positron, although there are many other particle antiparticle pairs described by the Dirac equation besides these. 1 2 Section 2 When we constructed the Dirac representation, we saw that it was the direct sum of two irreducible representations of the Lorentz group: 1 , 0 0, 1 . Now we see that it describes 2 ⊕ 2 two physically distinguishable particles: the electron and the positron. Irreducible unitary spin 1 2 representations of the Poincaré group, Weyl spinors, have 2 degrees of freedom. Dirac spinors have 4. These are 2 spin states for the electron and 2 spin states for the positron. For charged spinors, there is no other way. Uncharged spinors can be their own antiparticles if they are Majorana spinors, as discussed in Section 4 below. To understand the degrees of freedom within a 4-component Dirac spinor, first recall that in the Weyl basis, the γ matrices have the form 0 σ γ = µ (3) µ σ¯ 0 µ and the Lorentz generators S µν = i [ γµ , γν ] are block diagonal. Under an infinitesimal Lorentz 4 transformation 1 ( iθi βi) σi ψ ψ + − ψ (4) → 2 ( iθi + βi) σi In this basis, a Dirac spinor is a doublet of a left and a right-handed Weyl spinor ψ ψ = L (5) ψ R 1 , , 1 Here left-handed and right-handed refer to the 2 0 or 0 2 representations of the Lorentz group. The handedness of a spinor is also known as its chirality. It is helpful to be able to project out the left or right handed Weyl spinors from a Dirac spinor. We can do that with the γ5 matrix γ5 = iγ0 γ1 γ2 γ3 (6) In the Weyl representation 5 1 γ = − 1 , (7) so left- and right-handed spinors are eigenstates of γ5 with eigenvalues 1 . We can also define projection operators ± 1 + γ5 0 1 γ5 1 PR = = ,PL = − = (8) 2 1 2 0 2 2 which satisfy PR = PR and PL = PL and ψ 0 ψ ψ P L = ,P L = L (9) R ψ ψ L ψ 0 R R R 1 γ 5 Writing projectors as ± is useful because it is basis-independent. 2 It is easy to check that that ( γ5 ) 2 = 1 and γ5 , γµ = 0. Thus γ5 is like another gamma { } matrix, which is why we call it γ5 . This lets us formally extend the Clifford algebra to 5 genera- tors, γM = γ0,γ1 ,γ2 ,γ3,iγ5 so that γM ,γN = 2 gMN with gMN = diag(1 , 1 , 1 , 1 , 1) . If we were looking at representations{ of the 5-dimensional} Lorentz group, we− would− − use− this extended Clifford algebra. See [Polchinski] for a discussion of spinors in various dimensions. To understand the degrees of freedom in the spinor, let us focus on the free theory. In the Weyl basis, the Dirac equation is µ m iσ ∂µ ψL −µ = 0 (10) i σ¯ ∂µ m ψR − In Fourier space, this implies µ Q σ pµ ψR = ( E σQ p ) ψR = mψL (11) − · µ Q σ¯ pµ ψL = ( E + Qσ p ) ψL = mψR (12) · Chirality, helicity and spin 3 So the mass mixes the left and right handed states. Q σQ p In the absence of a mass, left and right handed states are eigenstates of the operator hˆ = · Qp | | with opposite eigenvalue, since E = Qp for massless particles. This operator projects the spin on the momentum direction. Spin projected| | on the direction of motion is called the helicity, so the left and right handed states have opposite helicity in the massless theory. When there is a mass, the left- and right-handed fields mix due to the equations of motion. However, since momentum and spin are good quantum numbers in the free theory, even with a mass, helicity is conserved as well. Therefore, helicity can still be a useful concept for the mas- sive theory. The distinction is that when there is a mass, helicity eigenstates are no longer the same as the chirality eigenstates ψL and ψR. By the way, the independent solutions to the free equations of motion for massless particles Q Q of any spin are the helicity eigenstates. For any spin, we will always find S Qp Ψ s = s p Ψ s , · 1 ± | | 1 Q Q Q where S = J are the rotation generators in the Lorentz group for spin s. For spin , S = σQ . 2 2 For spin 1, the rotation generators are given in Eqs. (15??) of the previous Lecture. For example, J3 has eigenvalues 1 with eigenstates (0, i, 1 , 0) and (0, i, 1 , 0) . These are the states of circularly polarized light± in the z direction, which are helicity− eigenstates. In general, the polarizations of massless particles with spin > 0 can always be taken to be helicity eigenstates. This is true for spin 1/2 and spin 1, as we have seen, it is also true for gravitons (spin 2), Rarita-Schwinger fields (spin 3/2) and spins s > 2 (although, as we proved in Lecture II-2, it is impossible to have interacting theories with massless fields of spin s > 2). We have seen that the left- and right-handed chirality states ψL and ψR do not mix under Lorentz transformations – they transform in separate irreducible repre- • sentations. each have two components on which the σQ matrices act. These are the two spin states of • the electron; both left- and right-handed spinors have 2 spin states. are eigenstates of helicity in the massless limit. • We have now seen 3 different spin-related quantities: Spin is a vector quantity. We say spin up, or spin down, spin left, etc. It is the eigenvalue of Qσ SQ = for a fermion. If there is no angular momentum, for example for a single particle, the spin 2 Q and the rotation operators are identical SQ = J . We also talk about spin s, as a scalar, which is 2 1 1 s s SQ s the eigenvalue ( + 1) of the operator . When we say spin 2 we mean = 2 . Helicity refers to the projection of spin on the direction of motion.
Details
-
File Typepdf
-
Upload Time-
-
Content LanguagesEnglish
-
Upload UserAnonymous/Not logged-in
-
File Pages13 Page
-
File Size-