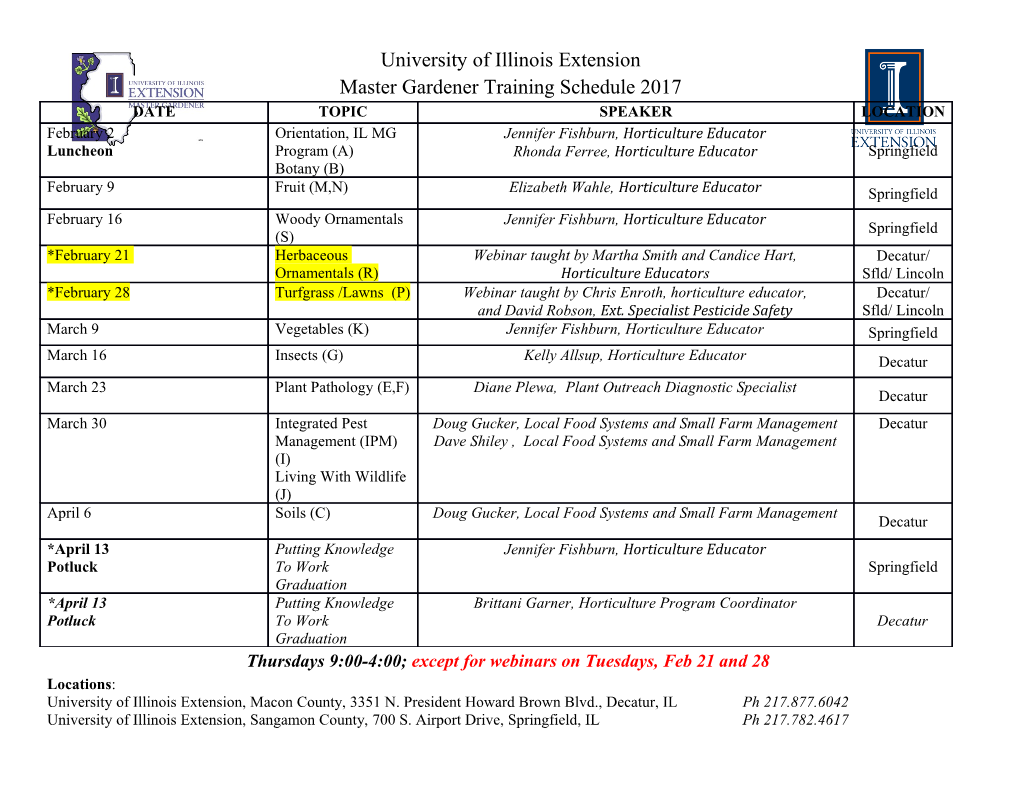
Photosynth Res DOI 10.1007/s11120-017-0364-0 REVIEW Photosynthetic fuel for heterologous enzymes: the role of electron carrier proteins Silas Busck Mellor1 · Konstantinos Vavitsas1 · Agnieszka Zygadlo Nielsen1 · Poul Erik Jensen1 Received: 3 October 2016 / Accepted: 27 February 2017 © Springer Science+Business Media Dordrecht 2017 Abstract Plants, cyanobacteria, and algae generate a sur- Introduction plus of redox power through photosynthesis, which makes them attractive for biotechnological exploitations. While Oxygenic photosynthesis uses the energy from absorbed central metabolism consumes most of the energy, pathways photons for the synthesis of ATP and the generation of introduced through metabolic engineering can also tap into reducing power in the form of NADPH. Most of this this source of reducing power. Recent work on the meta- energy is subsequently used to incorporate CO2 into tri- bolic engineering of photosynthetic organisms has shown ose phosphate molecules, which form the basis of anabolic that the electron carriers such as ferredoxin and flavodoxin and catabolic reactions. The core of photosynthetic energy can be used to couple heterologous enzymes to photo- metabolism, the conversion of light energy into chemi- synthetic reducing power. Because these proteins have a cal energy, has attracted research efforts from many disci- plethora of interaction partners and rely on electrostatically plines, and consequently the chemical and physical princi- steered complex formation, they form productive electron ples are known in considerable detail (Witt 1996; Nelson transfer complexes with non-native enzymes. A hand- and Yocum 2006; Umena et al. 2011; Mazor et al. 2015; ful of examples demonstrate channeling of photosynthetic Ago et al. 2016). electrons to drive the activity of heterologous enzymes, The electrochemistry of photosynthesis is unique in and these focus mainly on hydrogenases and cytochrome employing the most extreme redox potentials recorded in P450s. However, competition from native pathways and biological systems: that of the photo-oxidized photosystem inefficient electron transfer rates present major obstacles, II [Em= +1120 mV (Diner and Rappaport 2002)] and of which limit the productivity of heterologous reactions cou- the photo-excited photosystem I [Em= −1000 mV (Brettel pled to photosynthesis. We discuss specific approaches to 1997)]. Furthermore, the redox state of the photosynthetic address these bottlenecks and ensure high productivity of apparatus is a major regulator of cell metabolism in plants, such enzymes in a photosynthetic context. algae, and cyanobacteria, e.g., by controlling the light acti- vation of biosynthetic pathways via the ferredoxin/thiore- Keywords Ferredoxin · Flavodoxin · Synthetic biology · doxin, NADP/thioredoxin, and glutathione/glutaredoxin Metabolic engineering · Cytochrome P450 · Photosynthesis regulatory systems (Buchanan and Balmer 2005; Stenbaek and Jensen 2010; Yoshida and Hisabori 2016). Besides carbon fixation and redox regulation, cells chan- nel photosynthetic reducing power towards a multitude of biological processes, but it can also be directed towards * Poul Erik Jensen non-native enzyme activities. This review focuses on how [email protected] to exploit photosynthesis as a reductive driving force for heterologous reactions. We first introduce and briefly 1 Copenhagen Plant Science Center, Center for Synthetic Biology ‘bioSYNergy’, Department of Plant describe the major electron carrier proteins involved in and Environmental Sciences, University of Copenhagen, photosynthetic reactions and their interactions with partner Thorvaldsensvej 40, 1871 Frederiksberg C, Denmark enzymes. Next, we cover the work done using the electron Vol.:(0123456789)1 3 Photosynth Res carrier ferredoxin to direct reducing power towards the bio- electrons from photosystem I to ferredoxin–NADP+ reduc- synthesis of hydrogen and a handful of target chemicals. tase (FNR). As ferredoxin is a one-electron carrier, reduc- Finally, we discuss how redox coupling may be improved, tion of NADP+ to NADPH requires two sequential electron and why photosynthetic electron transport holds an impor- transfers from ferredoxin to FNR (Hanke and Mulo 2013). tant place in metabolic engineering and synthetic biology Ferredoxin–FNR and ferredoxin–photosystem I interac- applications. tions have been studied extensively through structural and kinetic techniques coupled with site-specific mutagenesis and involve prominent contributions from electrostatic Characteristics of electron carrier proteins interactions (Fig. 1) (Hurley et al. 2006; Sétif 2006). involved in photosynthetic electron transport Midpoint potentials of reduced ferredoxins range from −300 to −430 mV (Cammack et al. 1977), and those of Ferredoxin ferredoxins in photosynthetic electron transfer typically fall in the more negative end of this range. This permits the Photosynthetic electron transport requires small soluble reduction of NADP+ to NADPH (−320 mV) and allows electron carrier proteins to transfer reducing power from them to act as a hub distributing electrons towards central the cytochrome b 6f complex to photosystem I and from metabolic reactions in plants and cyanobacteria [reviewed photosystem I to various electron acceptors/acceptor pro- by (Hanke and Mulo 2013; Cassier-Chauvat and Chau- teins (Fig. 1). The latter is mostly carried out by ferre- vat 2014; Goss and Hanke 2014)]. Ferredoxin also plays doxins, which comprise a ubiquitous and highly diverse a key role in cyclic electron transport, which balances group of small, 6–13 kDa, iron–sulfur proteins, includ- the production of ATP and NADPH by recycling elec- ing 2Fe–2S, 3Fe–4S, 4Fe–4S, and single Fe (rubredoxin) trons through the plastoquinone pool and the cytochrome cluster configurations (Tagawa and Arnon 1968; Sticht b6f complex (Yamori and Shikanai 2016). Photosynthetic and Rösch 1998). Plant and cyanobacterial ferredoxins use organisms have several ferredoxin isoforms, with distinct a 2Fe–2S cluster coordinated by four conserved cysteines roles (Hanke et al. 2004; Cassier-Chauvat and Chauvat and act as the primary electron transfer protein for relaying 2014). Their central role in distributing reducing power Positive charge + NADP FNR Fld Negative charge NADPH Fd Stroma PQH2 PQ Lumen Photosystem I Cyt c Cytochrome b f 6 Photosystem II 6 H2O½O2 Pc Fig. 1 Schematic illustration of the photosynthetic electron transfer tion sites. Crystal structures used are as follows: photosystem II from apparatus in and around the thylakoid membrane. Arrows indicate Thermosynechococcus vulcanus (PDB: 4UB6), Cytochrome b6f from linear electron transport during photosynthetic activity, starting with Chlamydomonas reinhardtii (PDB: 1Q90), Cytochrome (Cyt) c6 the oxidation of water and ending with the reduction of NADP+ to from Synechococcus elongatus (PDB: 1C6S), plastocyanin (PC) from NADPH. A dashed arrow indicates back-donation of electrons via Populus nigra (PDB: 4DP1), photosystem I and ferredoxin–NADP+ the cytochrome b6f complex during cyclic electron transport. Pro- reductase (FNR) from Pisum sativum (PDB: 4Y28 and 1QG0, respec- teins are shown by their electrostatic surface potentials, which were tively), flavodoxin (Fld) fromSynechococcus sp. PCC 6301 (PDB: calculated using the APBS plugin (Baker et al. 2001) and visualized 1CZN), and ferredoxin (Fd) from Spinacia oleracea (PDB: 1A70). in PyMol. Red or blue dashed lines show the polarities of interac- PQ/PQH2 plastoquinone/plastoquinol 1 3 Photosynth Res derived from the photosynthetic apparatus, together with is absolutely necessary for photosynthetic growth in their negative redox potentials and innate ability to transfer land plants (Weigel et al. 2003), but it can be replaced by electrons to many different acceptor proteins, makes ferre- cytochrome c6 in cyanobacteria (Zhang et al. 1994; Clarke doxins the most explored electron donors for heterologous and Campbell 1996). redox enzymes, as will be described below. Cytochrome c6 proteins are small, 9–12 kDa, and diverse heme-containing proteins, found in electron trans- Flavodoxin port chains in prokaryotic and eukaryotic organisms, with redox potentials similar to that of plastocyanin (+320 mV Flavodoxins are functional homologs of ferredoxins and for Synechocystis sp. PCC 6803 c6) (Kerfeld and Krog- use flavin mononucleotide (FMN) as their redox cofactor. mann 1998; Cho et al. 1999; Howe et al. 2006). While most The flavodoxins are larger than ferredoxins, usually around cyanobacteria and green algae produce both plastocyanin 15–20 kDa, and are absent in plants, probably because fla- and cytochrome c6 (De La Rosa et al. 2002), some cyano- vodoxin genes of the ancestors of land plants were lost dur- bacteria (such as Arthrospira platensis) use cytochrome ing adaptation to iron-rich coastal environments (Karlusich c6 instead of plastocyanin (Sandmann 1986). Like plasto- et al. 2014). That said, some enzymes, e.g., diflavin reduc- cyanin, cytochrome c6 relies on electrostatic interactions tases, possess FMN-binding domains structurally homolo- for electron transfer, although the polarity of these can dif- gous to flavodoxins (Medina 2009), and the flavodoxin fer between plants and cyanobacteria (De La Rosa et al. fold, like the ferredoxin fold, is ancient and figures among 2002). Although a cytochrome c6 isoform was discovered the nine most ancient protein folds (Caetano-Anollés et al. in plants (Gupta et al. 2002; Wastl et al. 2002), it does not 2007). Despite the fact that
Details
-
File Typepdf
-
Upload Time-
-
Content LanguagesEnglish
-
Upload UserAnonymous/Not logged-in
-
File Pages14 Page
-
File Size-