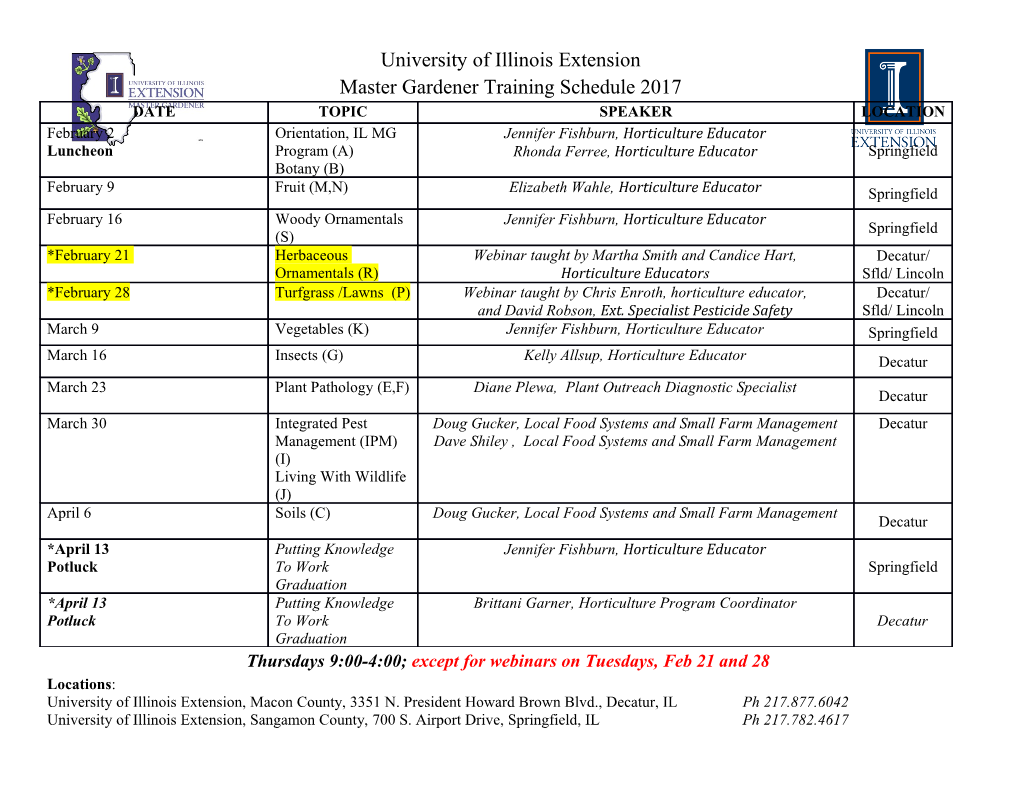
Earth and Planetary Science Letters, 80 (1986) 175-182 175 Elsevier Science Publishers B.V., Amsterdam - Printed in The Netherlands [61 Cratonization and thermal evolution of the mantle Henry N. Pollack Department of Geological Sciences, University of Michigan, Ann Arbor, MI 48109-1063 (U.S.A.) Received May 8, 1986; revised version received July 11, 1986 The stabilization of continental lithosphere to form cratons is accomplished by volatile loss from the upper mantle during magmatic events associated with the formation of continental crust. Volatile depletion elevates the solidus and increases the stiffness of the mantle residuum, thereby imparting a resistance to subsequent melting and deformation. Freeboard is maintained in part by the buoyancy associated with an increased Mg/(Mg + Fe) ratio in the mantle residuum following extraction of crustal material. Augmented subcratonic seismic velocities derive from the same shift in this ratio. The higher effective viscosity of the stabilized subcratonic upper mantle inhibits its entrainment in mantle convection, and locally thickens the conductive boundary layer. Heat approaching from greater depths is diverted away from the stiff craton to other areas that continue to transfer heat by convection, thus yielding a low surface heat flow within cratons. Cratonization by devolatilization and petrologic depletion was most effective in the Archean and has diminished in effectiveness over geologic time as the mantle temperature has fallen because of the declining store of internal heat. From the Archean to the present that ascending mantle material which has undergone partial melting has encountered the solidus at progressively shallower depth, has remained supersolidus over a smaller depth range, has temperatures which have exceeded the solidus by lesser amounts, has undergone diminishing degrees of partial melting, and has experienced less thorough devolatilization. At a given time the rate of production of continental crust is likely to be proportional to the depth extent and fraction of partial melting. Integration of the partial melt zone over time yields a growth curve that is similar to some continental crustal growth curves inferred from isotopic evolution. I. Introduction deformation and magmatism are clearly confined to the pre-stabilization history of the cratons, and The purpose of this paper is to set forth a marine incursions onto the stabilized cratons are model of the process by which continental litho- generally short-term transients. Cratons are truly sphere is stabilized to form cratons, and to ex- islands of stability within a generally mobile and amine how this process may have evolved through less stable lithosphere. geologic time. I use the term craton in its tradi- How do cratons acquire and maintain the prop- tional geologic sense as continental crust that has erties that give them long-term stability? As with attained stability and has been unaffected by oro- oceanic lithosphere, thermal thickening must be genic activity for a prolonged period of time. At one factor in the stabilization process. Early the same time, however, I make the case that the estimates of lithospheric thickness [1], based on development of cratonic stability is intimately as- surface heat flow and extrapolated geotherms, sociated with important changes in the sub- suggested that the cratonic lithosphere extends to cratonic mantle. depths of some 300 km. Jordan [2-4] has also The stability of cratons is characteristically dis- vigorously advocated thick root zones beneath the played in at least three ways: (1) resistance to continental cratons. The seismological evidence internal deformation, (2) a paucity of magmatism, advanced by him to substantiate the deep roots and (3) maintenance of freeboard. Of course one was debated for some time, but the issue now can cite exceptions to each of these, including seems resolved by recent complementary observa- extensional dyke swarms, anorogenic magmatism, tions [5-7] that confirm differences in seismic and marine supracrustal sedimentary sequences velocities, extending to 400 km depth, between the within cratons. However, large-scale and pervasive upper mantle beneath cratons and that beneath 0012-821x/86/$03.50 © 1986 Elsevier Science Publishers B.V. 176 non-cratonic parts of continents as well as oceans. PRESSURE (10 Spa) 20 40 60 80 100 120 Investigations of upper mantle electrical conduc- 2400 2400 tivity also indicate highly resistive roots extending 2000 to similar depths beneath cratons [8]. U 2000 Jordan has argued that thermal scenarios alone 1600 are inadequate to explain the stabilization of ,m c'~' , . SJ ~ cratonic lithosphere. He invokes a complementary ,,~ ~200 1200 stabilizing mechanism involving a distinctive G: buoyant composition for the cratonic root that would compensate for the increased density and 800 800 subsidence associated with the cooling of the lith- osphere following a major tectonothermal event. 400 peridotite solidus 400 His assessment that wholly thermal stabilization / i h i i models are inadequate is persuasive, and his ad- IO0 200 300 400 DEPTH (km) vancement of a compositional compensation mechanism has provided new insights into stabili- Fig. 1. Peridotite solidus in different volatile environments, zation by focussing on the petrological depletion after Wyllie [9,10]. Dashed line between CO 2 and H20 is processes associated with tectonothermal events. generalized solidus for a mixed volatile environment compris- ing equal amounts of CO 2 and H20. Also shown are character- However, even Jordan's fuller description of istic temperature differences between volatile-free and the stabilization process remains incomplete. A volatile-rich soliduses. significant element that must accompany the ther- mal and petrological aspects of stabilization is the depletion of volatiles from the sub-cratonic upper mantle. It is my opinion that devolatilization, par- less, than in a volatile-rich environment. Devola- ticularly associated with periods of significant tilization therefore elevates the solidus within the growth of continental crust in the early Pre- upper mantle, in effect making the mantle more cambrian, is a critical and essential aspect of the refractory and less vulnerable to subsequent melt- stabilization process. ing. Jordan [3] recognized that a garnet lherzolite which had undergone partial fusion and removal 2. Volatiles, melting, and deformation of components with low melting temperatures ("basalt depletion") would lead to a more refrac- Evidence that shows the significance of volatile tory residuum. However, the effect devolatiliza- depletion for stabilization derives from experi- tion has on elevating the solidus overshadows the ments on incipient melting, and on differential similar but lesser consequences of selective fusion. stress required to produce steady flow in rocks, Devolatilization has substantial effects on me- each performed in the presence and absence of chanical properties as well. Fig. 2 shows the gener- volatiles. Fig. 1 summarizes experimentally .ob- alized results of flow stress experiments on dunite served effects of the volatile environment on the referenced to a uniform strain rate, as a function solidus of peridotite [9,10]. The observational data of temperature and volatile environment [12-14]. on which Fig. 1 is based derives principally from The effect of devolatilization can be seen clearly: experiments below 5 GPa, although investigations the given strain rate can be achieved at the same [11] at pressures to 14 GPa are beginning to differential stress only if the temperature is constrain melting relations deeper within the up- elevated by some 450°C. Or, the strain rate can be per mantle. The effects are not exactly the same achieved at 1000°C only if the differential stress is for each volatile species, or for different volatile increased by almost two orders of magnitude, and ratios in a mixed-volatile environment. Neverthe- at 2000°C by one order of magnitude. Other less, the general effect of devolatilization is clear: experiments on polycrystalline olivine [15-17] incipient melting of mantle peridotite in a show some variation in the magnitude of strain volatile-free environment requires temperatures rate produced by a given differential stress, but 300-600°C higher, or pressures nearly 60 kbar consistently confirm the 1-2 orders of magnitude 177 log (o',-o%) (10SPa) sphere. The thickened boundary layer will be -3 -2 -1 0 2000 , , i r accompanied by a reduction in heat flow through it, and will lead to an adjustment in the convec- \ \ \ \ tion beneath and peripheral to it so as to maintain 1500 the integrated regional heat flux [19]. G That devolatilization has similar effects on both incipient melting and viscosity, i.e. requiring tem- peratures several hundred degrees higher in a volatile-free environment to achieve conditions and/or properties found in cooler volatile-rich 1000 settings, is a natural consequence of the fact that both melting and solid state creep are thermally activated processes, with activation energies for both substantially higher in a volatile-free environment. The amount of volatiles necessary to 750 reduce the activation energy is small; the esti- 19 20 21 22 LOG VISCOSITY (Poise) mated 0.1 wt.% H20 in the undepleted upper mantle [20] is certainly sufficient. Fig. 2. Steady-state flow stress in dunite at strain rate of 10- i4 s-I as a function of temperature in different volatile environ- 3. Process of cratonization ments, after Carter [14].
Details
-
File Typepdf
-
Upload Time-
-
Content LanguagesEnglish
-
Upload UserAnonymous/Not logged-in
-
File Pages8 Page
-
File Size-