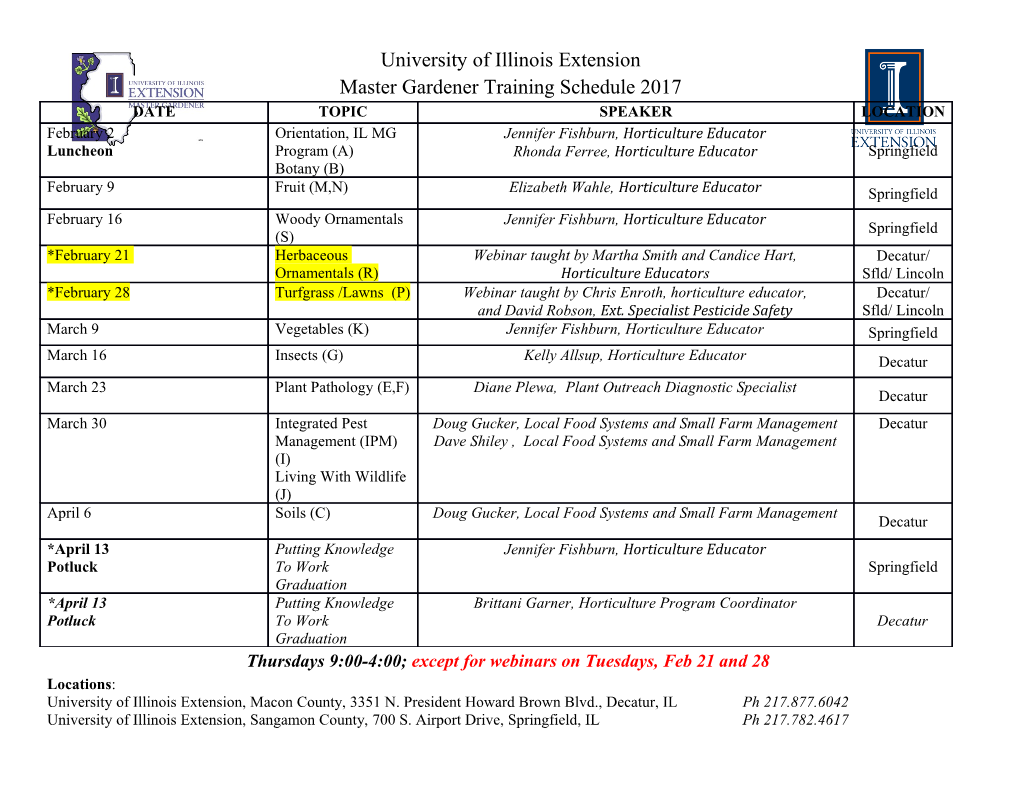
SnapShot: Auditory Transduction A.J. Hudspeth Howard Hughes Medical Institute and The Rockefeller University, 1230 York Avenue, New York, NY 10065, USA Traveling wave in normal animal Traveling wave in anoxic animal Cochlear cross-section Surface view, organ of Corti Vestibular Cochlear Stapes nerve nerve Scala vestibuli Incus Scala Malleus Outer hair cells T media Inner hair cells Scala tympani Tympanic External membrane Round window auditory (eardrum) Middle Organ of Corti canal Cochlea ear Tectorial membrane cavity Eustachian Inner hair tube Outer cell hair cell Endolymph Nerve bers Basilar membrane Perilymph Hearing plays a key role in alerting us to nearby activities, in the Stereocillia Kinocilium appreciation of music, Basal body and especially in Microvilli human communication. Cuticular Tight junction plate Airborne sounds vibrate the Belt desmosome tympanum, which transmits the oscillations successively to the malleus, incus, and stapes of the middle ear. Moving like a piston, the last of these miniscule bones Nucleus alternately increases and decreases the pressure within the cochlea, a chickpea-sized organ that is the SUPPORTING CELL most complex mechanical contrivance of the body—and the Basal lamina site of auditory transduction. Afferent nerve ending Efferent nerve ending See online version for 536 Neuron 80, October 16, 2013 ©2013 Elsevier Inc. DOI http://dx.doi.org/10.1016/j.neuron.2013.10.003 legends and references. SnapShot: Auditory Transduction A.J. Hudspeth Howard Hughes Medical Institute and The Rockefeller University, 1230 York Avenue, New York, NY 10065, USA The Cochlea and the Traveling Wave The human cochlea comprises three liquid-filled chambers, each slightly more than 30 mm in length, wound in three spiral turns about a common axis. The top cham- ber or scala vestibuli is attached to the stapes at the flexible oval window. The bottom chamber or scala tympani also has an orifice, the round window, sealed by a flexible membrane. The scala media lies between these two chambers, separated from the scala vestibuli by the thin Reissner’s membrane and from the scala tympani by the basilar membrane, which bears the elaborate sensory epithelium termed the organ of Corti. Auditory stimulation produces a fluctuating pressure difference between the scala vestibuli and scala tympani that vibrates the basilar membrane, initiating a traveling wave that propagates from the cochlear base toward the apex. The basilar membrane is narrow, taut, and light at the base but broad, slack, and massive at the apex. Owing to this gradation in the membrane’s properties, the wave grows in amplitude as it progresses, peaks at a particular position, and then decays abruptly. High-frequency signals culminate near the base, whereas low-frequency vibrations crest near the apex. The basilar membrane is thus a real-time frequency analyzer that decomposes a complex sound into its constituent frequencies and represents each as a vibration at a particular position. This characteristic allows us to discriminate an enormous variety of sounds by analyzing their frequency spectra. Transduction and Amplification by Hair Cells The human cochlea, like the hearing organs of other vertebrates, depends upon a single type of receptor: the hair cell. A cylindrical epithelial cell, the hair cell is distin- guished in every auditory organ of every vertebrate by a hair bundle extending 1–100 µm from its apical surface. This bundle comprises 10–300 upright, cylindrical, actin-filled stereocilia, enlarged microvilli that pivot at their bases. Acting through the mechanical apparatus of the organ of Corti, an acoustic stimulus applies oscillatory forces to the top of each hair bundle, driving the structure back and forth. This motion is transmitted through cadherin-based tip links to mechanically sensitive ion channels, probably of the TMC family, situated on the stereociliary tips. The opening and closing of these channels elicits a receptor potential that fosters the release of glutamate from ribbon synapses at the cell’s base and thus excites afferent axons. The hair cell is not a passive recipient of stimuli, but instead uses an active process to enhance its inputs. The active process amplifies mechanical stimuli by as much as a thousand-fold, greatly increasing our sensitivity to weak sounds. When this process fails, we become hard of hearing. Amplification is accompanied by frequency tuning. If the active process deteriorates, we grow less sensitive to subtle differences in frequency and suffer a diminished ability to discriminate sound sources. Finally, the active process produces a compressive nonlinearity that renders the ear sensitive to sounds over an astonishing trillion-fold range in power. By enhancing weak stimuli and suppressing strong ones, this feature allows us to enjoy an instrumental soloist as comfortably as a full orchestra one hundred times as loud—and to be offended by a crinkling candy wrapper of one-hundredth the intensity. The human cochlea contains hair cells of two types. A single row of 4,500 inner hair cells lies nearest the center of the cochlear spiral. These cells act as sensory transduc- ers, capturing stimulus energy, interpreting it as electrical responses, and forwarding the information to the brain. The 12,000 outer hair cells, which form three staggered rows, send little information into the brain; instead, they are responsible for implementing the active process. As a traveling wave advances along the basilar membrane, the active process in successive hair cells overcomes the inevitable loss of power owing to the viscosity of the moving liquids. As a consequence, the traveling wave’s magnitude is several-fold as large in a normal cochlea as in one in which the active process has been inactivated, for example by anoxia. The Basis of the Cochlear Active Process Two mechanisms cooperate in the active process of the mammalian cochlea. First, hair bundles serve not only as transducers but also as amplifiers. For sounds of rela- tively low frequency, myosin-1c motors attached at the upper insertions of tip links provide the motive force to enhance hair-bundle motion in response to weak stimuli. The active process can operate at frequencies approaching 100 kHz, however, a level seemingly exceeding the reaction rate of myosins. The molecular basis of active hair-bundle motility at such high frequencies remains uncertain. The other motile phenomenon, which performs most of the mechanical work in the mammalian cochlea, involves changes in the length of a hair cell’s entire soma. The plas- malemma of each outer hair cell is studded with millions of copies of the protein prestin, a modified anion transporter. Changes in potential alter the membrane area occupied by these molecules: depolarization shortens a hair cell whereas hyperpolarization elongates it. These periodic changes in length, a manifestation of somatic motility or electro- motility, pump energy into the basilar membrane’s oscillation. Although the membrane time constant limits the rate at which a hair cell’s potential can change, the amplifying effect of active hair-bundle motility evidently overcomes this restriction. The three cardinal features of the active process—amplification, frequency tuning, and compressive nonlinearity—emerge together in a dynamical system operating at an instability called the Hopf bifurcation. A bifurcation represents a qualitative change in a system in response to a continuous change in a control parameter. Traversing a Hopf bifurcation transforms an active but stable system into one that oscillates spontaneously. Remarkably enough, both individual hair bundles and the cochlea as a whole exhibit such instability. Under suitable conditions a hair bundle in vitro oscillates with metronomic regularity at the frequency to which it is tuned. And in quiet environments even normal human cochleas produce spontaneous otoacoustic emissions, one or more pure tones that actually emerge from the ear! ACKNOWLEDGMENTS Drs. P. Barr-Gillespie and A. Forge supplied electron micrographs. The author is an Investigator of Howard Hughes Medical Institute. REFERENCES Fisher, J.A.N., Nin, F., Reichenbach, T., Uthaiah, R.C., and Hudspeth, A.J. (2012). Neuron 76, 989–997. Hudspeth, A.J. (2008). Neuron 59, 530–545. Hudspeth, A.J., Jülicher, F., and Martin, P. (2010). J. Neurophysiol. 104, 1219–1229. Kawashima, Y., Géléoc, G.S., Kurima, K., Labay, V., Lelli, A., Asai, Y., Makishima, T., Wu, D.K., Della Santina, C.C., Holt, J.R., and Griffith, A.J. (2011). J. Clin. Invest. 121, 4796–4809. Kazmierczak, P., Sakaguchi, H., Tokita, J., Wilson-Kubalek, E.M., Milligan, R.A., Müller, U., and Kachar, B. (2007). Nature 449, 87–91. 536.e1 Neuron 80, October 16, 2013 ©2013 Elsevier Inc. DOI http://dx.doi.org/10.1016/j.neuron.2013.10.003.
Details
-
File Typepdf
-
Upload Time-
-
Content LanguagesEnglish
-
Upload UserAnonymous/Not logged-in
-
File Pages2 Page
-
File Size-