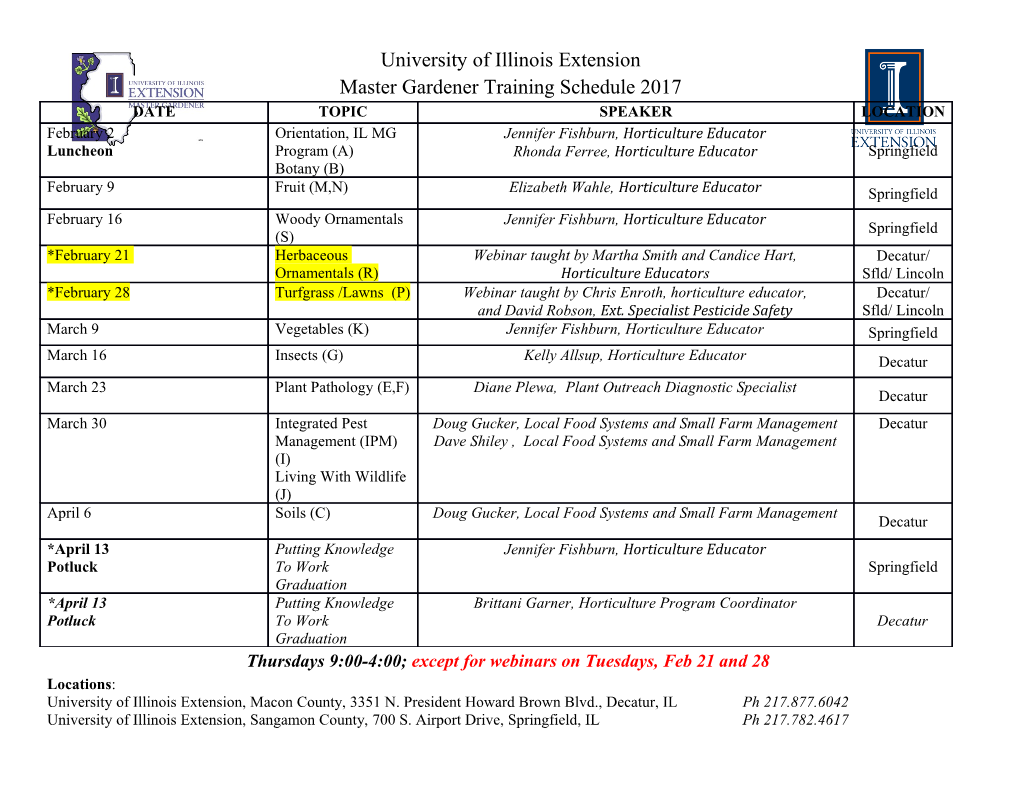
inorganics Review Structure: Function Studies of the Cytosolic, Mo- and NAD+-Dependent Formate Dehydrogenase from Cupriavidus necator Russ Hille 1,*, Tynan Young 2, Dimitri Niks 1, Sheron Hakopian 3, Timothy K. Tam 2, Xuejun Yu 4, Ashok Mulchandani 5 and Gregor M. Blaha 1,* 1 Department of Biochemistry, University of California, Riverside, CA 92521, USA; [email protected] 2 Department of Biochemistry and the Biochemistry and Molecular Biology Graduate Program, University of California, Riverside, CA 92521, USA; [email protected] (T.Y.); [email protected] (T.K.T.) 3 Department of Biochemistry and the Environmental Toxicology Graduate Program, University of California, Riverside, CA 92521, USA; [email protected] 4 Bioengineering Graduate Program, University of California, Riverside, CA 92521, USA; [email protected] 5 Department of Chemical and Environmental Engineering, University of California, Riverside, CA 92521, USA; [email protected] * Correspondence: [email protected] (R.H.); [email protected] (G.M.B.); Tel.: +1-951-827-6354 (R.H.); +1-951-827-4294 (G.M.B.) Received: 19 May 2020; Accepted: 28 June 2020; Published: 6 July 2020 Abstract: Here, we report recent progress our laboratories have made in understanding the maturation and reaction mechanism of the cytosolic and NAD+-dependent formate dehydrogenase from Cupriavidus necator. Our recent work has established that the enzyme is fully capable of catalyzing the reverse of the physiological reaction, namely, the reduction of CO2 to formate using NADH as a source of reducing equivalents. The steady-state kinetic parameters in the forward and reverse directions are consistent with the expected Haldane relationship. The addition of an NADH-regenerating system consisting of glucose and glucose dehydrogenase increases the yield of formate approximately 10-fold. This work points to possible ways of optimizing the reverse of the enzyme’s physiological reaction with commercial potential as an effective means of CO2 remediation. New insight into the maturation of the enzyme comes from the recently reported structure of the FdhD sulfurase. In E. coli, FdhD transfers a catalytically essential sulfur to the maturing molybdenum cofactor prior to insertion into the apoenzyme of formate dehydrogenase FdhF, which has high sequence similarity to the molybdenum-containing domain of the C. necator FdsA. The FdhD structure suggests that the molybdenum cofactor may first be transferred from the sulfurase to the C-terminal cap domain of apo formate dehydrogenase, rather than being transferred directly to the body of the apoenzyme. Closing of the cap domain over the body of the enzymes delivers the Mo-cofactor into the active site, completing the maturation of formate dehydrogenase. The structural and kinetic characterization of the NADH reduction of the FdsBG subcomplex of the enzyme provides further insights in reversing of the formate dehydrogenase reaction. Most notably, we observe the transient formation of a neutral semiquinone FMNH , a species that has not been observed previously with holoenzyme. After initial · 1 reduction of the FMN of FdsB by NADH to the hydroquinone (with a kred of 680 s− and Kd of 190 µM), one electron is rapidly transferred to the Fe S cluster of FdsG, leaving FMNH . The Fe S cluster of 2 2 · 4 4 FdsB does not become reduced in the process. These results provide insight into the function not only of the C. necator formate dehydrogenase but also of other members of the NADH dehydrogenase superfamily of enzymes to which it belongs. Keywords: nicotinamide adenine dinucleotide (NADH); electron transfer; enzyme kinetics; enzyme structure; formate dehydrogenase; carbon assimilation Inorganics 2020, 8, 41; doi:10.3390/inorganics8070041 www.mdpi.com/journal/inorganics Inorganics 2020, 8, 41 2 of 13 1. Introduction The molybdenum- and tungsten-dependent formate dehydrogenases have drawn increased attention over the past 5–10 years due to the demonstration that under the appropriate conditions, most, if not all, are able to catalyze the reverse reaction, reduction of CO2 to formate, under the appropriate conditions. Indeed, some enzymes of this family, which include the closely related formylmethanofuran dehydrogenases of the Wood–Ljungdahl pathway, function physiologically to reduce CO2 to formate in what is probably the most evolutionarily ancient mechanism of carbon fixation. Cupriavidus necator H16 (previously known as Ralstonia eutropha) has four formate dehydrogenases, of which, two are cytosolic enzymes that utilize NAD+ as oxidizing substrate [1,2]. One of these contains molybdenum and is encoded by the fdsGBACD operon, the other possesses tungsten and is encoded by the fdwAB operon (presumably enlisting additional subunits from the fds operon) [3]. The molybdenum-containing enzyme was originally isolated and characterized by Bowien and coworkers, who showed that the mature holoenzyme belongs to the NADH dehydrogenase superfamily of enzymes [4–6]. The FdsA, FdsB, and FdsG subunits are homologous to corresponding subunits in the cytosolic arm of NADH dehydrogenase [7–10]. FdsA is homologous to the Nqo3 subunit of the NADH dehydrogenase from Thermus thermophilus [8,9], although the latter lacks a molybdenum center as found in the former [7]. The homology between FdsA and Nqo3 includes the presence of a histidine ligand to one of the Fe4S4 clusters. The C-terminal domain of FdsA, containing the molybdenum center, is also homologous to the crystallographically characterized FdhF formate dehydrogenase of E. coli, with the molybdenum-coordinating Cys 378 of FdsA equivalent to Sec 140 in FdhF [11]. The Fe4S4- and FMN-containing FdsB subunit is homologous to the T. thermophilus Nqo1 subunit and like Nqo1 also possesses a binding site for NADH/NAD+. The FdsG subunit is homologous to the T. thermophilus Nqo2 subunit and has a single Fe2S2 cluster. We report here recent work from our laboratories on both mechanistic and structural aspects of the C. necator enzyme. 2. Catalysis of CO2 Reduction by the C. necator Formate Dehydrogenase Under physiological conditions, reducing equivalents enter the C. necator formate dehydrogenase holoenzyme at its molybdenum center and leave at the FMN, reducing NAD+ to NADH; electron transfer between the molybdenum and flavin, which are separated by some 55 Å [10], is mediated by the intervening iron–sulfur clusters. Although originally reported to be unable to catalyze the CO2 by NADH, we have recently shown that the enzyme is indeed capable of doing so when CO2 (not bicarbonate) is used as substrate [12]. This is consistent with a mechanism for formate VI VI oxidation involving direct hydride transfer of the Cα-H to the Mo = S group of the L2Mo S(S-Cys) molybdenum center (where L is the bidentate enedithiolate-coordinated pyranopterin cofactor found in molybdenum- and tungsten-containing enzymes, present in this enzyme as the guanine dinucleotide) IV to L2Mo (SH)(S-Cys), with CO2 rather than bicarbonate as the immediate product of the reaction [13]. The steady-state kinetic parameters have been determined in both the forward and reverse directions and are shown in Table1. Table 1. Steady-state kinetic parameters for the C. necator formate dehydrogenase. Forward Reverse 1 1 kcat 201 s− 10 s− formate Km 130 µM– NAD+ Km 310 µM– CO2 Km – 2700 µM NADH Km – 46 µM Inorganics 2020, 8, 41 3 of 13 The Haldane relationship for these parameters for an enzyme, such as formate dehydrogenase Inorganics 2020, 8, x FOR PEER REVIEW 3 of 14 operating via a ping-pong mechanism with separate sites for the reductive and oxidative half-reactions, is as follows:The Haldane relationship for these parameters for an enzyme, such as formate dehydrogenase forward forward (k ) (k ) 1 1 operating via a ping-pong mechanismcat cat with separate(201 ssites− ) (for201 the s− ) reductive and oxidative half- formate NAD+ reactions, is as follows: (Km ) · (Km ) (130 µM) (310 µM) K = = · = 1250 (1) eq reverse reverse ( 1) ( 1) (kcat) (kcat) 10 s− 10 s− k k 201 s 201 s CO ∙ NADH (2700 µM) ∙(46 µM) KK2 ·(KmK) 130 μM· 310 μM K = m = = 1250 k k 10 s 10 s (1) ∙ ∙ The value 1250 compares favorably withK the Keq2700calculated μM 46 from μM the 100 mV difference between K + DE00 for the NAD /NADH and CO2/formate couples of 2100, the disparity reflecting a ~10% uncertainty forwardThe value 1250reverse compares favorably with the Keq calculated from the 100 mV difference between in kcat and kcat , which are squared terms in numerator and denominator, respectively, ΔE0′ for the NAD+/NADH and CO2/formate couples of 2100, the disparity reflecting a ~10% of Equationuncertainty (1). in kcatforward and kcatreverse, which are squared terms in numerator and denominator, Therespectively, reaction can of Equation be pushed (1). significantly in the direction of CO2 reduction by the addition of an NADH regenerationThe reaction system can be [ 14pushed]. As significantly shown in Figure in the 1direction left, addition of CO2 reduction of a catalytic by the amount addition of of formatean NADH regeneration system [14]. As shown in Figure 1 left, addition of a catalytic amount of formate dehydrogenase to a solution that is 29.5 mM in CO2 (at 30 ◦C) and 300 µM in NADH results in the formationdehydrogenase of 120 µM formateto a solution (as that quantified is 29.5 mM by in ion CO chromatography)2 (at 30 °C) and 300 µM and in 130 NADHµM results NAD+ in—in the other formation of 120 µM formate (as quantified by ion chromatography) and 130 µM NAD+—in other words, the reaction is tightly coupled. When the experiment is repeated with the addition of 50 mM words, the reaction is tightly coupled. When the experiment is repeated with the addition of 50 mM glucoseglucose and a catalyticand a catalytic amount amount of glucose of glucose dehydrogenase dehydrogenase for NADH NADH regeneration, regeneration, the amount the amount of of formateformate generated generated increases increases approximately approximately 10-fold 10-fold toto 1.01.0 mM mM (Figure (Figure 1 1right).
Details
-
File Typepdf
-
Upload Time-
-
Content LanguagesEnglish
-
Upload UserAnonymous/Not logged-in
-
File Pages13 Page
-
File Size-