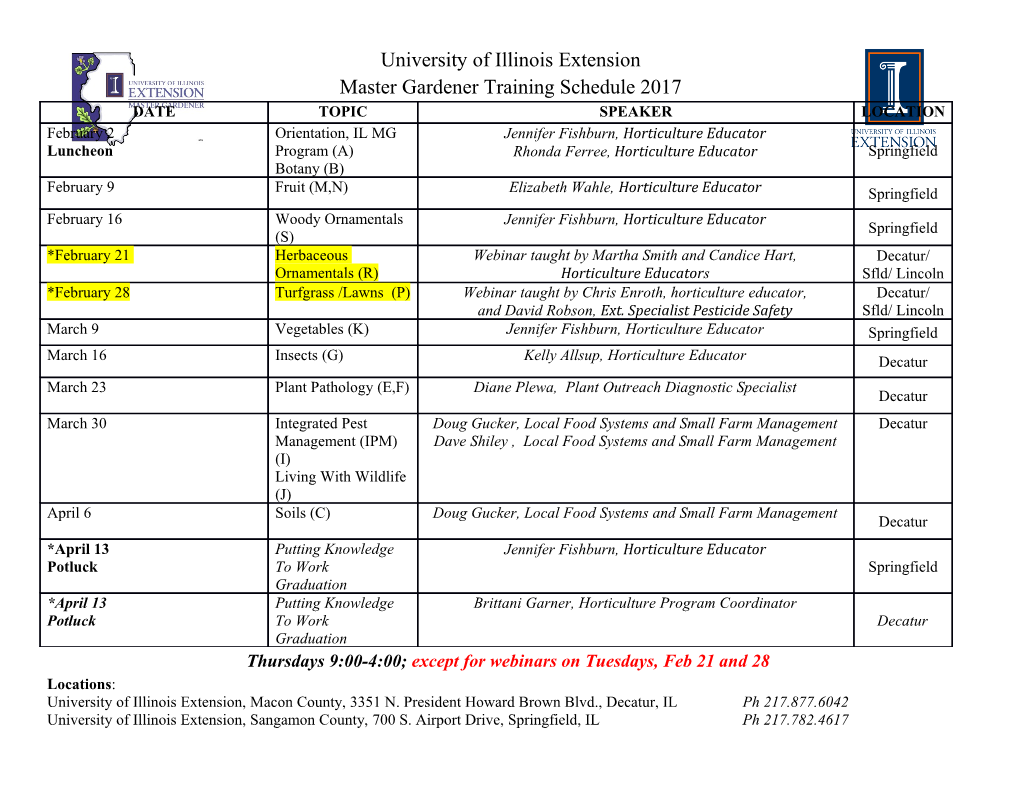
Hydrate Risk Assessment and Restart- Procedure Optimization of an Offshore Well Using a Transient Hydrate Prediction Model L.E. Zerpa, E.D. Sloan, C.A. Koh, and A.K. Sum, Colorado School of Mines Summary This paper focuses on the application of the gas-hydrate model A produced-hydrocarbon stream from a wellhead encounters for- to study the hydrate-plugging risk of an offshore well with a typ- mation of solid gas-hydrate deposits, which plug flowlines and ical geometry and fluid properties from the Caratinga field located which are one of the most challenging problems in deep subsea fa- in the Campos basin, Brazil. Three different periods of the well cilities. This paper describes a gas-hydrate model for oil-dominated life are considered: an early stage with low production gas/oil ratio systems, which can be used for the design and optimization of facil- (GOR) and low water cut, a middle stage with an increased GOR, ities focusing on the prevention, management, and remediation of and a late stage with higher GOR and higher water cut. The hy- hydrates in flowlines. Using a typical geometry and fluid properties drate-plugging risk is estimated from the calculation of three per- of an offshore well from the Caratinga field located in the Campos formance measures (pressure drop along flowline, hydrate volume basin in Brazil, the gas-hydrate model is applied to study the hy- fraction in pipe, and hydrate-slurry relative viscosity) in an attempt drate-plugging risk at three different periods of the well life. Addi- to quantify the plugging risk. Once the hydrate-plugging risk is tionally, the gas-hydrate model is applied to study the performance estimated for the three different periods of the well life, the gas- of the injection of ethanol as a thermodynamic hydrate inhibitor in hydrate model is used to optimize the concentration of thermody- steady-state flow and transient shut-in/restart operations. The ap- namic hydrate inhibitor (THI) to be injected in order to reach a safe plication of the transient gas-hydrate model proved to be useful in operation condition. In this study, ethanol is considered as the THI determining the optimal ethanol concentration that minimized the because it is the typical hydrate inhibitor used in South America hydrate-plugging risk. (because of the large industrial production of ethanol in the region). Additionally, the gas-hydrate model is used to study the effect Introduction of hydrates in transient shut-in/restart operations of the offshore Offshore explorations in deeper and colder waters impose more- well and the performance of hydrate inhibition in terms of ethanol challenging scenarios to the flow assurance of the produced concentration. In general, ethanol injection decreased the hydrate- streams. High pressures and low temperatures of operation of pro- plugging risk in steady-state and transient operations. The appli- duction facilities with longer subsea tiebacks will promote the for- cation of the transient gas-hydrate-prediction model proved to be mation of natural-gas hydrates: crystalline compounds formed by useful in determining the optimal ethanol concentration that mini- hydrogen-bonded water molecules in a lattice structure that is sta- mized the hydrate-plugging risk. bilized by encapsulating a small guest molecule (e.g., methane and ethane) (Sloan and Koh 2008). Gas hydrates form in the pres- Description of the Hydrate Prediction Model ence of appropriate quantities of gas and water, and are considered The hydrate-prediction model was specially designed for oil-dom- one of the most challenging problems in deep subsea facilities be- inated systems on the basis of the conceptual model presented in cause of their rapid formation compared with other solid deposits Fig. 1, which represents an approximation to the mechanism of hy- (Sloan 2005). drate-plug formation and is divided into four main stages. A transient gas-hydrate model that predicts when and where hy- 1. Water entrainment: The water droplets are dispersed in the drate plugs will form in flowlines will have significant utility for the continuous oil phase as a water-in-oil emulsion. flow-assurance engineer in the oil and gas industry. By predicting gas- 2. Hydrate growth: A hydrate shell forms at the interface be- hydrate formation and transportability, the gas-hydrate model can be tween the water droplets and the surrounding oil phase. applied to design and optimize oil/gas-transport facilities, focusing on 3. Agglomeration: The hydrate-encrusted water droplets can ag- prevention, management, or remediation of gas hydrates in flowlines. glomerate, increasing into larger hydrate masses or agglomerates. This paper briefly presents a gas-hydrate model specially designed 4. Plugging: The agglomeration of hydrate particles leads to an for oil-dominated systems, which has been incorporated as a plug-in increase in the slurry viscosity, which can eventually result in a module in a transient multiphase-flow simulator (Turner et al. 2005). plug. The gas-hydrate model developed for oil-dominated systems follows The model assumes that all the water is dispersed into the contin- the conceptual model presented in Fig. 1, where hydrates form at the uous oil phase as water droplets of fixed diameter, where the surface interface of water droplets entrained in the continuous oil phase. In area between water and hydrocarbon phases is calculated by a tran- the oil phase, these hydrate-encrusted water droplets can agglomerate sient multiphase-flow simulator using the Hinze correlation (Hinze into larger hydrate masses, leading to an increase in the slurry vis- 1955). Hydrate nucleation is assumed to occur immediately after cosity, which can eventually result in a plug (Turner 2006). a specified subcooling (DTsub=Thyd_eq-Tsystem), and the hydrate growth is calculated using the following intrinsic kinetics equation of first order with an adjustable rate constant (Boxall 2009), Copyright © 2012 Society of Petroleum Engineers This paper (SPE 160578) was revised for publication from paper OTC 22406, first presented at the Offshore Technology Conference Brasil, Rio de Janeiro, 4–6 October 2011. Original dmgas k2 manuscript received for review 14 September 2011. Revised manuscript received for review =−uk1 exp. ATs ()∆ sub .......................................... (1) 1 December 2011. Paper peer approved 9 February 2012. dt Tsys PB Oil and Gas Facilities • October 2012 October 2012 • Oil and Gas Facilities 49 Water Entrainment Hydrate Growth Agglomeration Plugging Gas Oil Water Fig. 1—Conceptual model for hydrate formation in multiphase-flow systems consisting of water, oil, and gas; adapted from Turner (2006). 0 TABLE 1—PROPERTIES AND COMPOSITION OF Platform CARATINGA CRUDE OIL (SJÖBLOM ET AL. 2010) 500 Wellhead Density (g/m3) 0.914 1000 Viscosity dead oil (Pa·s) 0.262 Viscosity live oil (Pa·s) 0.0095 1500 IFT (mN/m) 23 Depth (m) 2000 Asphaltene content (%) 6.2 Well Saturates content (%) 39.8 2500 Flowline and riser Aromatic content (%) 39.8 3000 Resin content (%) 14.3 0 5 10 15 Horizontal Distance (km) Then, the relative viscosity of the hydrate slurry to the viscosity of the continuous oil phase is calculated using Mills’ equation Fig. 2—Geometry of well, flowline, and riser, based on typical (Mills 1985): geometries from the Caratinga field located at the Campos Ba- sin in Brazil. 1− φeff µr = 2 . ................................................................... (4) The intrinsic kinetics equation gives the rate of gas consump- φ 1− eff tion during hydrate formation as a function of the intrinsic rate φmax constants (k1 and k2) regressed from the data of Vysniauskas and Bishnoi (1983), the surface area (As) between water and hydro- A hydrate plug is identified by a large viscosity increase, which carbon phases, and the subcooling as the thermal driving force. causes an unacceptably large pressure drop in the line, prohibiting flow. Once the hydrate particle has formed, the size of the agglomer- ates is calculated using a steady-state balance between the interpar- Offshore-Well Hydrate Risk Assessment ticle cohesion force and the shear forces (Camargo and Palermo This section presents the approach followed to estimate the hy- 2002), assuming a constant interparticle cohesion force as follows: drate-plugging risk in an offshore well using the gas-hydrate-pre- diction model described in the preceding section. The simulation case consists of a typical well/flowline/riser geometry and fluid 3− f 2 φ d properties from the Caratinga field located at the Campos Basin F 1− hyd A 4− f a in Brazil. Fig. 2 shows the geometry in terms of horizontal length d φmax dP A − = 0, ............................... (2) and depth from sea level, which consists of a slanted well with a di- 3− f dP ameter of 5 in., a departure of 2000 m, and a depth of 1800 m. The 2 d A dPµγ0 1− φhhyd well is connected to a straight flowline with a slight uphill slope, d P a diameter of 0.1524 m, and a horizontal length of approximately 13 km, followed by the bend into the riser that leads up to the plat- where dA is the hydrate-agglomerate diameter, dP is the hydrate- form or FPSO. Table 1 presents a summary of the Caratinga crude- particle diameter, fhyd is the hydrate-particle volume fraction, fmax oil properties and composition measured by Sjöblom et al. (2010). is the maximum packing fraction (assumed to be equal to 4/7), f is As a first approach to estimate hydrate-plugging risk, a pressure/ the fractal dimension (assumed to be equal to 2.5) that accounts for temperature diagram, shown in Fig. 3, was built overlying the flow- the porosity of the aggregates, Fa is the interparticle cohesion force line operation conditions under steady-state flow with hydrate-equi- (assumed to be equal to 50 mN/m), m0 is the oil viscosity, and γ is librium curves at different ethanol concentrations.
Details
-
File Typepdf
-
Upload Time-
-
Content LanguagesEnglish
-
Upload UserAnonymous/Not logged-in
-
File Pages8 Page
-
File Size-