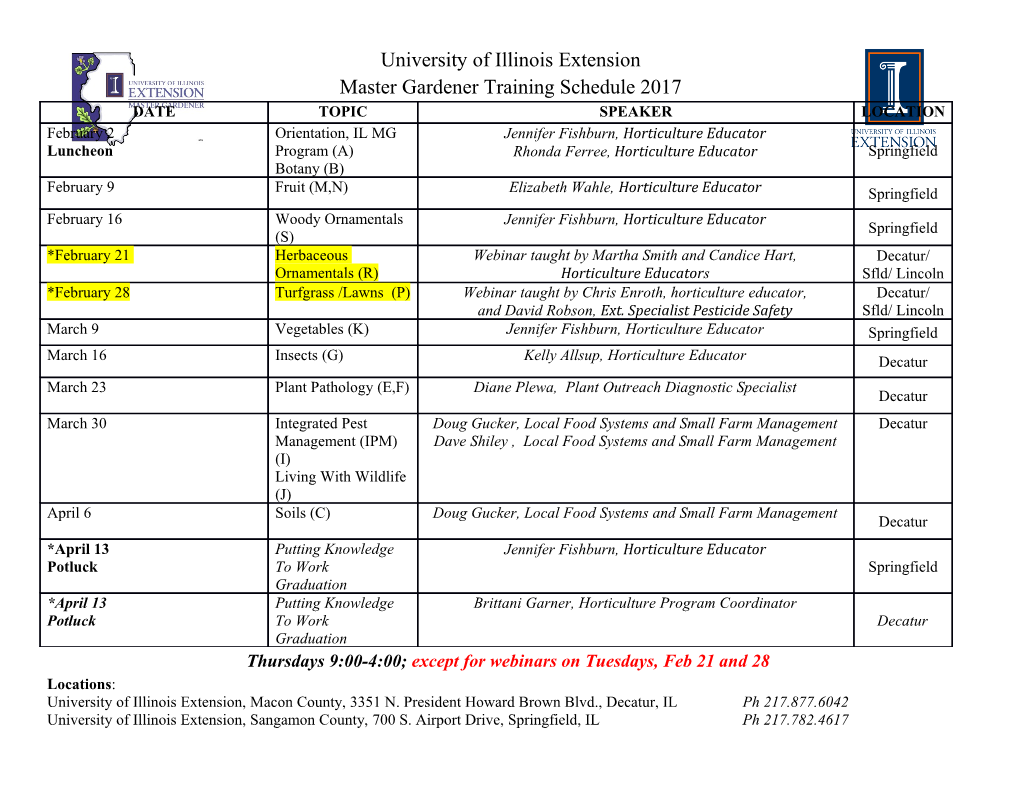
Characterization of Iron Loading in the Hamp Knockout Rat Savannah Nelson CALS Honors Program Honors Thesis Spring 2019 Food Science and Human Nutrition Department College of Agricultural and Life Sciences Faculty Advisor: Dr. James F. Collins 1 TABLE OF CONTENTS Abstract…………………………………………………………………………………………...3 Introduction………………………………………………………………………………………4 Materials and Methods…………………………………………………………………………..7 Animal Experiments……………………………………………………………………..7 Non-Heme Iron Measurements…………………………………………………………8 Tissue Histology………………………………………………………………………….8 Statistical Analysis……………………………………………………………………….8 Results…………………………………………………………………………………………….9 Non-Heme Iron Measurements…………………………………………………………9 Tissue Histology………………………………………………………………………….9 Discussion…………………………………………………………………………………….....10 Literature Cited………………………………………………………………………………...13 Supporting Figures/Tables……………………………………………………………………..16 Figure 1: Non-Heme Tissue Iron Levels………………………………………………16 Figure 2: Tissue Histology……………………………………………………………...18 Figure 3: Late-Stage Tissue Histology………………………………………………...20 2 ABSTRACT Iron is an essential mineral for several physiological functions. Since humans are unable to excrete iron, intestinal iron absorption must be tightly controlled by the hormone hepcidin. Hepcidin agonists or mimetics offer attractive pharmaceutical targets for treating iron overload conditions. Previous research in our lab has shown that hepcidin (Hamp) ablation in rats causes iron overload, like that observed in human Type IIb hereditary hemochromatosis, by 9 weeks of age. However, the exact onset and progression of tissue iron accumulation is unknown. This study aimed to characterize iron loading between 3 and 9 weeks of age. Hamp-/- rats and wildtype controls (Hamp+/+) were sacrificed at 3, 4.5, 6, 7.5, and 9 weeks. Non-heme iron levels in the liver, heart, kidney, spleen, and pancreas were assessed by a colorimetric assay. Perls’ Prussian blue tissue staining revealed the location of iron deposition. Iron levels gradually -/- increased in Hamp rats in the liver, starting at 4.5 weeks of age (females) and 6 weeks (males). Iron status in wildtype controls remained relatively constant. With the determination of iron loading onset in the Hamp-/- rats, implications include the development and testing of therapeutic strategies in this pre-clinical rat model to reduce or prevent iron loading. 3 INTRODUCTION Iron is a required micronutrient for humans and other mammals as it is used for multiple important processes such as cellular growth, energy metabolism, and the delivery of oxygen to tissues (Gulec, Anderson, & Collins, 2014). Iron is present in the diet associated with organic molecules such as heme (i.e. heme iron) (present in animal products) or in its free, ferric state as non-heme iron (found in plant and meat sources). Absorption of both types of dietary iron is highly regulated at the upper part of the small intestine due to the lack of an excretory mechanism. Non-heme iron absorption is important to study because it is more commonly found in food but is more difficult for the body to absorb (Milto, Suhodolo, Prokopieva, & Klimenteva, 2016). Many proteins regulate iron oxidation, reduction, and transport as it passes through the apical (top) and basolateral (bottom) membranes of enterocytes, the cells of the small intestine. This ferric iron carries a +3 charge and must be reduced to ferrous iron with a +2 charge before it is transported into the enterocyte by a protein called divalent metal-ion transporter. Inside the cell during times of low demand, the storage protein ferritin can store iron (Gulec et al., 2014). This iron can then be transported across the basolateral membrane and into the bloodstream via the transmembrane glycoprotein ferroportin, the only known mammalian iron exporter (Milto et al., 2016). Ferroportin is in turn regulated by the peptide hormone hepcidin, derived from the liver. Hepcidin can block iron flow into the bloodstream by binding to ferroportin, causing it to be internalized and degraded by lysosomes inside the cell (Nemeth et al., 2004). Once degraded, ferroportin can no longer export iron, so the net effect is a decrease in the release of absorbed iron into the blood. In the absence of hepcidin, ferroportin is fully functional, so iron can be released from intestinal enterocytes, and macrophages and, hepatocytes (which store excess iron) 4 and support various physiological functions, including, most importantly erythropoiesis in the bone marrow. Thus, it is seen that hepcidin is down-regulated in times of high demand for iron (such as during erythropoiesis, when heme is synthesized and incorporated in red blood cells for oxygen transport), while it is upregulated when body iron stores are excessive (Milto et al., 2016). Mutations in hepcidin affecting its expression can lead to iron loading disorders in humans and other mammals. In humans, mutations in the HAMP (hepcidin antimicrobial peptide) gene can cause multi-organ iron overload in a condition known as Type IIb juvenile hereditary hemochromatosis (HH). This can lead to organ damage in the liver, heart, and other organs since the lack of hepcidin allows iron to escape enterocytes and migrate to these organs. HH may also present itself comparable to other endocrine disorders such as diabetes and liver cirrhosis (Pietrangelo, 2010) or cause iron-deficiency anemia, which is harmful during periods of growth (Gehrke et al., 2003). Studies investigating hepcidin ablation in mice have found similar consequences of systemic iron overload; however, mice may not represent a complete model for human hereditary hemochromatosis since mice are unaffected by certain endocrine disorders (Flores et al, 2017). Therefore, a rat model of this disease is warranted. Novel hepcidin knockout Sprague-Dawley (outbred) rats have been developed at the University of Florida to assess the regulation of iron metabolism and are only available in the laboratory of Dr. James Collins. These rats do not express hepcidin, so they are observed to develop iron overload in various organs due to the unregulated and excessive absorption of dietary iron. They represent a newly developed model of hereditary hemochromatosis (Flores et al., 2017). 5 It is known that these hepcidin knockout rats have normal body iron status at 3 weeks of age and become iron-loaded by 9 weeks of age (Flores et al., 2017). However, the point at which iron loading begins in each organ and its progression has yet to be investigated. This is significant because such information could be used in this pre-clinical, rat model of hereditary hemochromatosis as a prelude to human testing of alternatives to hepcidin for iron metabolism. We hypothesize that hepcidin knockout rats will begin over-loading iron in the liver, heart, and pancreas soon after weaning as they begin to consume solid food, which contains more iron than the breast milk they were previously consuming. 6 MATERIALS AND METHODS Animal Experiments All animal studies were approved by the University of Florida IACUC and performed upholding the guidelines of the American Association for Laboratory Animal Sciences. A breeding colony of hepcidin (whole-body) knockout (Hamp-/-) Sprague-Dawley rats was already established at the University of Florida, from which heterozygous (Hamp+/-) animals were provided. Hamp+/- rats were interbred, from which the offspring were genotyped for selection of knockout and wildtype (Hamp+/+) rats. All pups were housed in the UF Food Science and Human Nutrition Animal Care Facility during the study. All animals were fed an iron- adequate, AIN-93G-based diet (Reeves, Nielson, & Fahey, 1993) and deionized tap water with ad libitum access. Previous research indicating iron loading by 9 weeks of age led to the selection of a 6- week time period to assess the progression of iron accumulation. Starting at weaning (3 weeks of age) and occurring at ages of 4.5, 6, 7.5 and 9 weeks of age, 10 Hamp-/- rats (5 female, 5 male) +/+ and 10 littermate controls (Hamp ) were sacrificed via CO2 narcosis and subsequent thoracotomy. Whole liver, kidney, pancreas, spleen, and heart samples were collected. All remaining tissues were held in -80°C storage until further analysis. Blood was collected from the posterior vena cava to determine hemoglobin (Hb) and hematocrit (Hct) levels. A HemoCue Hb analyzer (Hemocue AB) and a Readacrit Hct system (BD Sedi-CalTM) were used to measure these parameters, respectively, following manufacturers’ instructions. 7 Non-Heme Iron Measurement To determine organ nonheme iron status at each time point, samples from the liver, kidney, heart, pancreas, and spleen were digested in acid solution (3M HCl and 10% trichloroacetic acid) overnight. Samples were analyzed by a colorimetric assay (chromogen- based) to quantify nonheme iron levels, adapted from a previously established method (Torrance & Bothwell, 1968). Tissue Histology Small tissue samples from each organ were submitted for histology to the University of Florida Molecular Pathology Core to assess the location of iron loading within the liver, pancreas, heart, kidney, and spleen. Tissue sections mounted to slides were stained using Perl’s Prussian Blue staining method (Perls, 1867), then enhanced with DAB peroxidase substrate solution to visualize iron deposits in these tissues. Tissue slides were scanned at the Univeristy of Florida Molecular Pathology Core. Images were viewed using Aperio ImageScope software (Aperio ImageScope 12.4 for Windows 10, Leica Biosystems Imaging,
Details
-
File Typepdf
-
Upload Time-
-
Content LanguagesEnglish
-
Upload UserAnonymous/Not logged-in
-
File Pages20 Page
-
File Size-