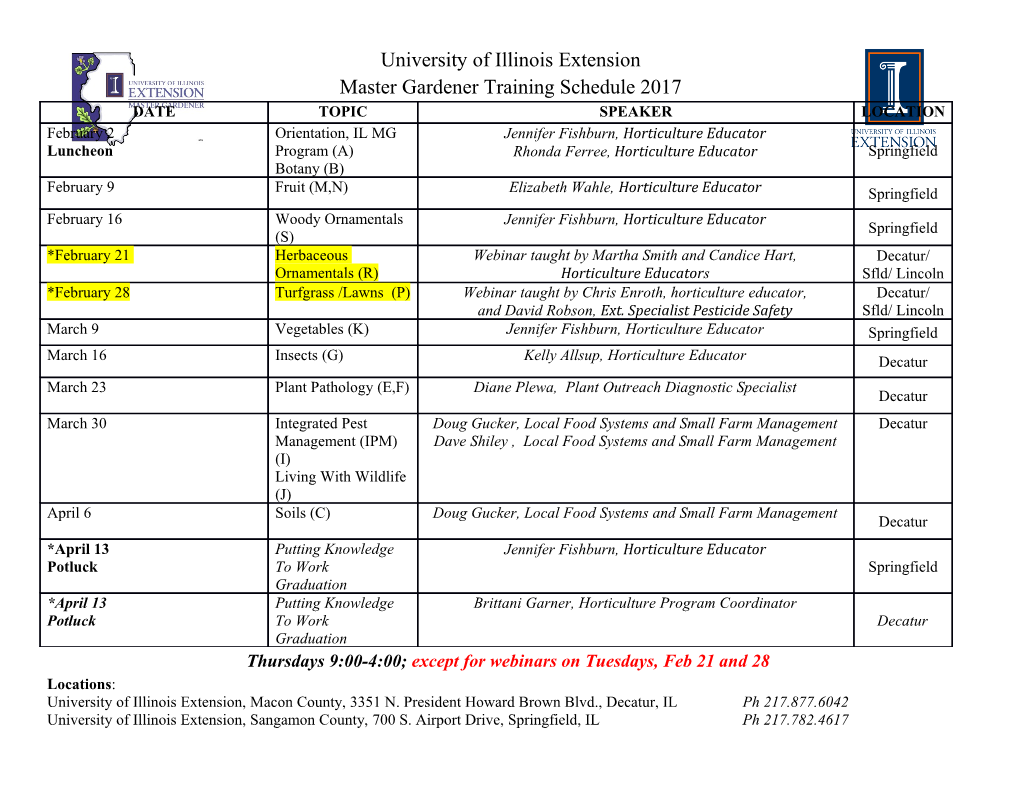
BULLETIN (New Series) OF THE AMERICAN MATHEMATICAL SOCIETY Volume 42, Number 4, Pages 549–554 S 0273-0979(05)01067-0 Article electronically published on July 1, 2005 Introduction to M¨obius differential geometry, by Udo Hertrich-Jeromin, London Mathematical Society Lecture Notes Series, vol. 300, Cambridge University Press, Cambridge, UK, 2003, xi+413 pp., US$50.00, ISBN 0-521-53569-7 M¨obius differential geometry is a classical subject that was extensively developed in the nineteenth and early twentieth centuries, culminating with the publication of Blaschke’s Vorlesungenuber ¨ Differentialgeometrie III: Differentialgeometrie der Kreise und Kugeln [2] in 1929. Research in the field was less intense over the next fifty years, but a resurgence of activity began about 1980, leading to some of the most interesting developments in submanifold theory over the past 25 years. The book under review lays the groundwork for both the classical and modern developments in the theory, leading the reader to the frontiers of research on several topics. M¨obius (conformal) differential geometry grew out of the classical study of curves and surfaces in 3-dimensional Euclidean space R3.InM¨obius geometry, there is measurement of angles but no measurement of lengths, and one searches for geo- metric quantities that are invariant under the group of conformal transformations of the ambient space. It is possible to study M¨obius geometry of surfaces in R3 by placing the usual Euclidean metric on R3 and trying to find Euclidean geometric quantities which are invariant under the group of conformal transformations of R3. However, this is often cumbersome, and it is not the classical approach of Blaschke’s book nor of the book under review. We begin by describing this classical approach in some detail and later will briefly describe two modern formulations of the theory which are also developed in this book. The classical model of M¨obius geometry is situated in the context of projec- tive geometry. Since Rn is conformally equivalent via stereographic projection to Sn −{p},whereSn is the unit sphere in Rn+1 and p ∈ Sn isthepoleofthestere- ographic projection, one can use Sn instead of Rn as the base space in the study of conformal geometry. This is often simpler, since Sn is compact, and there is no need to consider an improper point ∞ nor to distinguish between hyperspheres and hyperplanes. The construction of the classical model begins by embedding Rn+1 as an affine subspace of real projective space RP n+1 as follows. Consider the Lorentz space n+2 R1 with Lorentz inner product (1) x, y = −x0y0 + x1y1 + ...+ xn+1yn+1, n+2 ∈ n+2 for x =(x0,x1,...,xn+1),y =(y0,y1,...,yn+1)inR1 . A vector x R1 is said to be spacelike, timelike or lightlike, respectively, depending on whether x, x n+1 n+2 is positive, negative or zero. The set L of all lightlike vectors in R1 ,givenby the equation 2 2 2 (2) x0 = x1 + ...+ xn+1, forms a cone of revolution called the light cone. Timelike vectors are “inside the cone” and spacelike vectors are “outside the cone”. Now consider the real projective n+1 n+2 ∼ ∼ space RP = R1 / , where x y if x is a non-zero scalar multiple of y,and 2000 Mathematics Subject Classification. Primary 53A30. c 2005 American Mathematical Society Reverts to public domain 28 years from publication 549 550 BOOK REVIEWS ∈ n+2 n+1 let [x] denote the projective equivalence class of the vector x R1 .InRP , n+1 one can embed R as the affine space of points [(x0,x1,...,xn+1)] with x0 =0 . Each such point has a unique representative of the form [(1,y1,...,yn+1)] = [(1,y)], n+1 with yi = xi/x0, for 1 ≤ i ≤ n +1, and y ∈ R . Note that for x =(1,y), one has (3) x, x = (1,y), (1,y) = −1+y · y, where y · y is the usual Euclidean inner product on Rn+1.Thusx, x =0ifand only if y · y =1, that is, y ∈ Sn. So the set Ln+1/ ∼ of projective classes of lightlike n+2 n n+1 points in R1 is diffeomorphic to the unit sphere S in R . Objects of fundamental importance in M¨obius geometry are the hyperspheres in Sn. Consider the hypersphere S in Sn with center p and spherical radius ρ, 0 <ρ<π.The hypersphere S is the intersection of Sn with the hyperplane in Rn+1 given by the equation (4) p · y =cosρ, 0 <ρ<π. If we let [(1,y)] be a point in the affine space, then (4) can be rewritten as (5) (1,y), (cos ρ, p) =0. Thus a point y ∈ Sn is in the hyperplane given by (4) if and only if [(1,y)] lies in the polar hyperplane in RP n+1 of the point (6) [ξ]=[(cosρ, p)]. Note that (7) ξ,ξ = − cos2 ρ + p · p =1− cos2 ρ =sin2 ρ>0, n+2 for 0 <ρ<π,sothatξ is a spacelike vector in R1 . Thus, the hypersphere S given by equation (4) can be identified with the spacelike point [ξ]inRP n+1.So the space of hyperspheres in Sn is diffeomorphic to the “outer space” of spacelike points in projective space, n+1 { | } (8) RPO = [ξ] ξ,ξ > 0 . Note that [ξ]=[ζ], where ζ = ξ/ ± sin ρ and ζ,ζ =1. Thus, the Lorentz sphere, n+1 { ∈ n+2| } (9) S1 = ζ R1 ζ,ζ =1 , n+1 is a double cover of RPO . Note that one can distinguish the two possible ori- entations of the sphere S by assigning a plus or minus sign to the radius ρ.This leads to Lie’s geometry of oriented spheres, which is actually the point of view of Blaschke’s book (see also [4] and [10]), in which M¨obius geometry is considered as a subgeometry of Lie sphere geometry. By a direct calculation, one can show that the two hyperspheres S1 and S2 cor- responding to the projective points [ξ1]and[ξ2], respectively, intersect orthogonally if and only if (10) ξ1,ξ2 =0, and one can determine the angle of intersection between any two spheres by a similar formula involving the Lorentz inner product. By definition, a M¨obius transformation is a diffeomorphism µ : Sn → Sn that maps hyperspheres to hyperspheres. Thus, a M¨obius transformation induces a map n+1 from the space of spheres RPO to itself, and using the Fundamental Theorem of Projective Geometry, one can show that this transformation naturally extends to a unique projective transformation on RP n+1.Inthisway,aM¨obius transformation BOOK REVIEWS 551 can be identified with a projective transformation on RP n+1 that maps the space Sn = Ln+1/ ∼ onto itself. One can then prove that such a projective transforma- ∈ n+2 tion must be induced by an orthogonal transformation A O1(n +2)of R1 . Such a projective transformation must preserve the polarity condition (10) and therefore preserve angles between hyperspheres, so it corresponds to a conformal transformation of Sn. Conversely, Liouville’s Theorem states that if φ : U → V is a conformal diffeomorphism between connected open subsets U and V in Sn,n≥ 3, then there is a unique M¨obius transformation µ such that φ is the restriction of µ to U. We now discuss the study of submanifolds in M¨obius geometry. Basically, sub- manifolds are considered as envelopes of differentiable families of spheres. To make this precise, one defines a sphere congruence to be a differentiable m-parameter m → n+1 m → n family of spheres S : M RPO . A differentiable map f : M S is called an envelope of a sphere congruence S if for all p ∈ M m, m (11) f(p) ∈ S(p)andTf(p)f(M ) ⊂ Tf(p)S(p), m m where Tf(p)f(M )andTf(p)S(p) denote the tangent subspaces to f(M )andS(p), respectively, at the point f(p). Neither f nor S is required to be an immersion, although such conditions are often assumed. One can formulate the enveloping conditions (11) in terms of the Lorentz inner product as follows. First, one knows that f,f =0,sincef maps into Sn. Further, recalling that the Lorentz sphere n+1 n+1 S1 is a double cover of RPO , one can locally choose homogeneous coordinates for the sphere congruence S so that S, S = 1. Then one can easily show that in this situation, the two conditions in (11) become, respectively, (12) f,S =0anddf , S =0. Furthermore, differentiating the condition f,S =0andusingdf , S =0,one obtains (13) f,dS =0. Thus, one can interpret this in two different ways. One can consider S as a spacelike unit normal field for the mapping f, or one can consider f as a lightlike normal field for the mapping S. Both points of view are important and are used to prove m → n+1 m → n+1 important results in the book. A pair of maps f : M L and S : M S1 that satisfy (12) is called a strip, and strips are the basic setup for local differential geometry in M¨obius geometry. An important concept in M¨obius differential geometry is the notion of the central sphere congruence of an immersion. Specifically, given an immersion f : M n−1 → n n−1 → n+1 S , there is precisely one sphere congruence Z : M S1 such that the corre- sponding shape operator (Weingarten tensor) AZ determined by Z as a normal field of f has trace zero.
Details
-
File Typepdf
-
Upload Time-
-
Content LanguagesEnglish
-
Upload UserAnonymous/Not logged-in
-
File Pages6 Page
-
File Size-