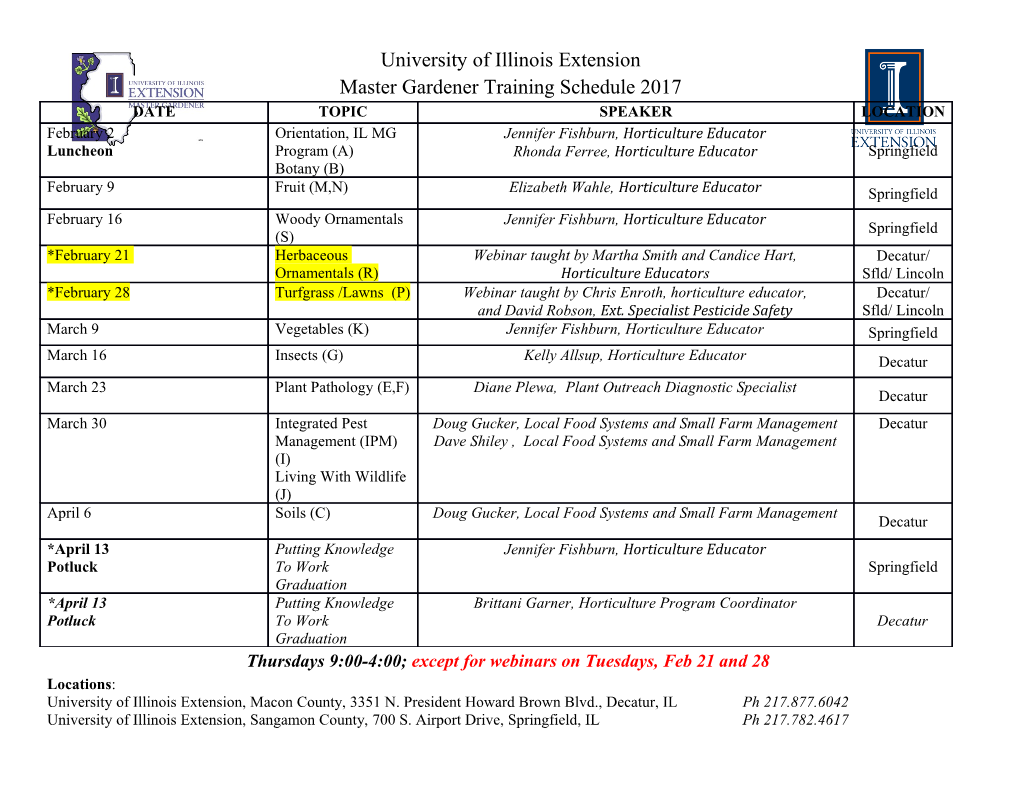
Cystatin: a protein that flips out! Protease inhibitors are vital in many biological processes which rely on preventing or controlling the degradation of proteins. The cystatin family of inhibitors bind tightly but reversibly to cysteine proteases, i.e. those which have a cysteine at their active site, in order to inhibit their activity. Cystatins are present in most higher organisms, from potatoes to people, where they control rates of protein turnover and combat parasitic and viral invasion. Cystatin superfamily Members of the cystatin superfamily share a common fold: an α­helix cradled in a sheet of five antiparallel β­strands (view­1). The superfamily can be divided into three major families. Type 1 cystatins, also called Stefins (e.g., PDB entries 1nb5, 1cyu), are found inside cells where they inhibit lysosomal proteases such as cathepsins. They are typically around 100 amino­acid residues in length. Type 2 cystatins (e.g., PDB entries 1cew, 1rn7) are slightly longer than the type 1 form, having an insertion between strands three and four. They are around 120 residues long, contain disulfide bonds and may be glycosylated. Type 2 cystatins are secreted and inhibit proteases found in the extracellular fluid. The kininogens make up the third cystatin family; they contain multiple cystatin domains within the same polypeptide chain. Disease implications In humans, cystatins are implicated in a variety of diseases. Deficiency in Stefin B, a type 1 cystatin, is implicated in the neurological disorder myoclonus epilepsy, whilst a lack of the type 2 cystatin C leads to the thinning of arteries and ultimately aneurism. One of cystatin C’s roles is to regulate the proteases that degrade the extracellular matrix, so without it artery walls can become thin and burst. Excess cystatin C is implicated in diseases such as atherosclerosis, diabetes, obesity, coronary heart disease, stroke, cerebral haemorrhage and dementia. The level of cystatin C in the bloodstream is used clinically as a marker of kidney function; raised levels suggest that the kidneys are not working optimally. Complex wedge Cystatins form tight but reversible complexes with their target proteases. Structures of such complexes (e.g., PDB entries 1stf, 1yvb, 3kse) show that they bind in the active site of the protease using what has been termed a ‘tripartite wedge’. This consists of a wedge­shaped group of mostly hydrophobic residues that lie at one edge of the β­sheet (view­2) but arise from three regions of the protein sequence, namely the N­terminus and the two loops between the β­strands. These residues insert into the active­site groove of the protease, blocking access to substrate proteins. The shape of this wedge is complementary to that of the groove, which contributes to the tight binding. Plot strain Loop 1 in cystatins, between β­strands two and three, is a tight turn containing a conserved three­residue motif of valine­X­glycine where X is usually a small amino acid such as alanine or serine. The hydrogen­bond ladder between strands two and three extends all the way to pdbe.org the valine in the loop, which forms a hydrogen bond with the glycine two residues along in the motif (view­3). This holds the valine in an unusually strained conformation. This unusual conformation enables cystatin to fit into the active site of cysteine proteases and may also help prevent the inhibitor becoming a substrate of those proteases. Such strain is rare in protein structures, but when observed, almost always occurs in regions critical for function (ref. 1). The strain in this valine is not induced by binding to the protease, nor is it an artefact of crystallisation. Both crystal and NMR structures (e.g., PDB entry 1dvd) of free cystatins also show this strain. A phi­psi plot of the backbone torsion angles (Fig. 1) for this valine highlights its unusual conformation. The plot shows that this combination of torsion angles is energetically unfavourable and rarely observed in protein structures. Fig 1. Phi­psi plot for valine 57, the strained residue in human cystatin C. Red regions represent favourable backbone torsion­angle combinations for valine residues, as defined by MolProbity, and yellow regions represent allowed angle combinations. The labels α, β, αL and γ indicate regions in which α­helices, β­strands, left­handed helices and γ­turns are found respectively. Valine 57 (green circles) lies in an unfavoured region of the plot when the protein is monomeric (V57M) but moves to the β­strand region when domain­swapping occurs (V57DS), as explained in the text. One good turn While the strained turn results in a good fit of the inhibitor with its target protease, it is also the protein’s Achilles’ heel. The conformation of this residue is geometrically and energetically unfavourable. Like the spring in a set mouse­trap, it can rotate and release its stored energy. As a result, the cystatin monomer is somewhat unstable and is frequently observed in a more stable, but inactive form (e.g., PDB entries 1g96, 1n9j). The stable form is one where the molecular spring has sprung, alleviating the strain in the valine conformation and moving it to a favourable position on the phi­psi plot where residues in β­strands are typically found (Fig. 1). In this form, the tight turn of loop 1 no longer exists and a long, continuous β­strand is formed, destroying the protease­binding surface and thus inactivating the inhibitor (view­4). The process flips out the helix and the first two strands of cystatin, opening up the whole structure and exposing many hydrophobic residues to the surrounding solvent. This structure is not stable on its own as the core of the protein has been destroyed but another cystatin molecule which has also ‘flipped out’ can interact such pdbe.org that the monomer fold, and its hydrophobic core, is restored but is now formed from two different protein chains with an intermolecular β­sheet. This phenomenon is known as domain swapping. This domain­swapped form of cystatin has been observed for both type 1 and type 2 cystatins, and even for plant cystatins (e.g., PDB entries 3ul5, 3ul6). Making fibres Once the domain swap has occurred, the cystatin dimers are able to oligomerise further. Stefin B forms tetramers (PDB entry 2oct), whilst crystals of human cystatin C can have an octameric assembly (e.g., PDB entry 1r4c). Ultimately, cystatins aggregate to form proteinaceous deposits called amyloid although the mechanism by which this occurs is not fully understood. Amyloid is an insoluble, fibrous protein deposit which can be formed by many different proteins and is implicated in diseases such as dementia, stroke and brain haemorrhage. The Icelandic mutation A mutation in the cystatin C gene found in some Icelandic families leads to a fatal condition called hereditary cystatin C amyloid angiopathy (ref. 2). In this disease, amyloid deposits are laid down in the blood vessels of the brain and other tissues. Symptoms include brain haemorrhage, stroke, dementia and death before the age of forty. The mutation involves a leucine at position 68 being swapped for a glutamine. This residue is buried deep within the hydrophobic core of the protein between the helix and the sheet (view­5). Placing a polar (and slightly bulkier) glutamine at this position greatly destabilises the monomer. The molecule is even more likely to ‘flip out’ as the hydrophobic core which holds the molecule together is weakened. In effect, the mutation makes the mousetrap much more sensitive and more likely to be sprung, leading to dimers and higher aggregates that cause the observed pathology. A measure of structural quality The phi­psi plot, often called the Ramachandran plot after one of the authors of the first paper (ref. 3) to define such plots of favourable backbone torsion­angle combinations, is used by structural biologists to assess the quality of a structure model. Usually, all protein residues would be expected to lie in the favourable regions of the phi­psi plot. Residues in unfavourable regions of the phi­psi plot merit closer inspection (of the model and the experimental data). It may simply mean that the model was built incorrectly, or, in cases like the cystatin family, there may be a good functional reason why a residue adopts an unusual conformation. References. Ref. 1 Herzberg O & Moult J (1991) Analysis of the steric strain in the polypeptide backbone of protein molecules. Proteins 11 223­9. Ref. 2 Palsdottir A et al. (2006) Hereditary cystatin C amyloid angiopathy: genetic, clinical, and pathological aspects. Brain Pathol. 16 55­9. pdbe.org Ref. 3 Ramachandran GN et al. (1963) Stereochemistry of polypeptide chain configurations. J. Mol. Biol. 7 95­9. Views. View 1: Structure of Cystatin. Stefin A, a type 1 cystatin is initially shown in cartoon reresentation with green α­helices and gold β­strands. Cystatin C, a type 2 cystatin, is then shown in the same colours. The insertion between strands two and three is coloured navy and the disulfide bonds present in type 2 cystatins are shown as sticks. Finally, the two structures are shown superposed with the type 1 and type 2 structures coloured light blue and dark green respectively. View 2: Cystatin binds to cysteine proteases. Cystatin C (dark green cartoons) is shown bound to Falcipain­2 (dodgerblue cartoons and aquamarine surface), a protease from the malarial parasite Plasmodium falciparum. The active site cysteine is shown in navy and the cystatin residues in the tripartite wedge which bind to falcipain are shown as salmon sticks. The conserved valine in loop 1 is highlighted in magenta. Finally, the cystatin surface is shown in orchid.
Details
-
File Typepdf
-
Upload Time-
-
Content LanguagesEnglish
-
Upload UserAnonymous/Not logged-in
-
File Pages6 Page
-
File Size-