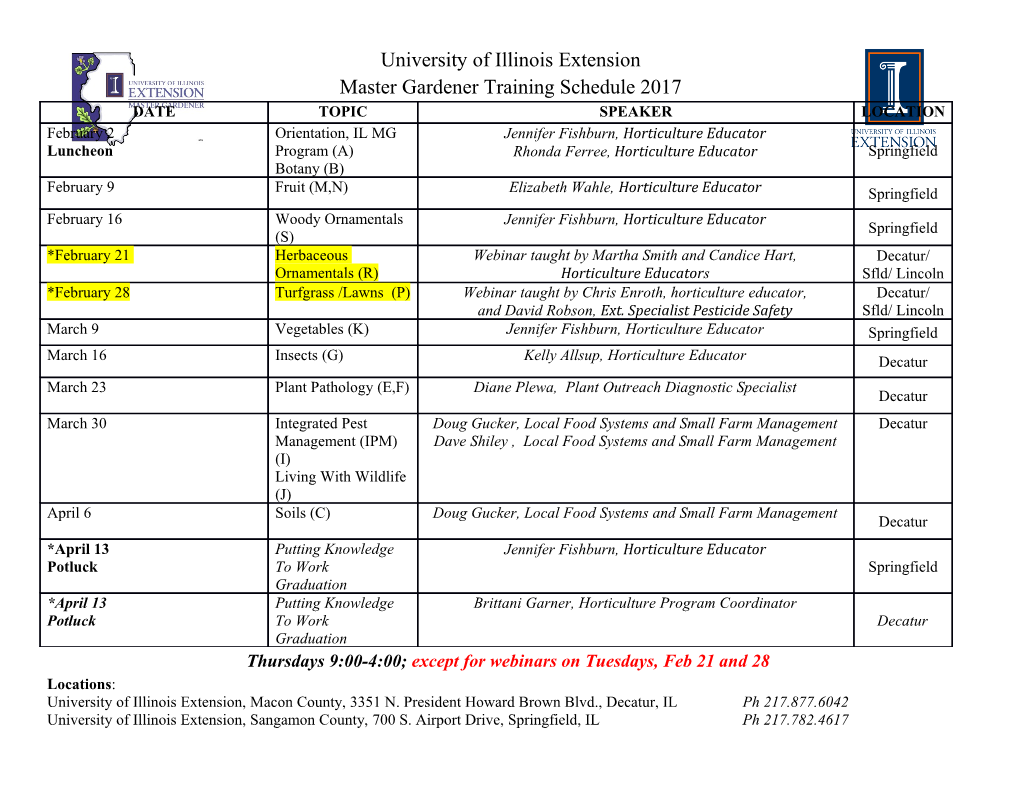
catalysts Article Description of Transport Tunnel in Haloalkane Dehalogenase Variant LinB D147C+L177C from Sphingobium japonicum Iuliia Iermak 1, Oksana Degtjarik 2, Petra Havlickova 3, Michal Kuty 3, Radka Chaloupkova 4, Jiri Damborsky 4,5, Tatyana Prudnikova 3,* and Ivana Kuta Smatanova 3,* 1 Department of Structural Cell Biology, Max Planck Institute of Biochemistry, Am Klopferspitz 18, 82152 Martinsried, Germany; [email protected] 2 Department of Structural Biology, Weizmann Institute of Science, Rehovot 7610001, Israel; [email protected] 3 Faculty of Science, University of South Bohemia in Ceske Budejovice, Branisovska 1760, 37005 Ceske Budejovice, Czech Republic; [email protected] (P.H.); [email protected] (M.K.) 4 Loschmidt Laboratories, Department of Experimental Biology and RECETOX, Faculty of Science, Masaryk University, Kamenice 5, 62500 Brno, Czech Republic; [email protected] (R.C.); [email protected] (J.D.) 5 International Clinical Research Center, St. Anne’s University Hospital Brno, Pekarska 53, 65691 Brno, Czech Republic * Correspondence: [email protected] (T.P.); [email protected] (I.K.S.) Abstract: The activity of enzymes with active sites buried inside their protein core highly depends on the efficient transport of substrates and products between the active site and the bulk solvent. The engineering of access tunnels in order to increase or decrease catalytic activity and specificity in a rational way is a challenging task. Here, we describe a combined experimental and computational approach to characterize the structural basis of altered activity in the haloalkane dehalogenase LinB Citation: Iermak, I.; Degtjarik, O.; D147C+L177C variant. While the overall protein fold is similar to the wild type enzyme and the Havlickova, P.; Kuty, M.; Chaloupkova, other LinB variants, the access tunnels have been altered by introduced cysteines that were expected R.; Damborsky, J.; Prudnikova, T.; Kuta Smatanova, I. Description of to form a disulfide bond. Surprisingly, the mutations have allowed several conformations of the Transport Tunnel in Haloalkane amino acid chain in their vicinity, interfering with the structural analysis of the mutant by X-ray Dehalogenase Variant LinB crystallography. The duration required for the growing of protein crystals changed from days to D147C+L177C from Sphingobium 1.5 years by introducing the substitutions. The haloalkane dehalogenase LinB D147C+L177C variant japonicum. Catalysts 2021, 11, 5. crystal structure was solved to 1.15 Å resolution, characterized and deposited to Protein Data Bank https://dx.doi.org/10.3390/catal11 under PDB ID 6s06. 010005 Keywords: bacterial enzyme; haloalkane dehalogenase; mutant form; crystallization; tertiary struc- Received: 20 November 2020 ture; disulfide bond; protein engineering; molecular dynamics; access tunnel; substrate specificity Accepted: 18 December 2020 Published: 23 December 2020 Publisher’s Note: MDPI stays neu- 1. Introduction tral with regard to jurisdictional claims in published maps and institutional The members of the haloalkane dehalogenase family (EC 3.8.1.5) are responsible affiliations. for the cleavage of the carbon–halogen bond in various halogenated compounds, one of the key reactions in the aerobic degradation of halogenated environmental pollutants [1]. Haloalkane dehalogenase LinB from a bacterium Sphingobium japonicum UT26 is able to convert cyclic dienes, broad range of halogenated alkanes and alkenes to their correspond- Copyright: © 2020 by the authors. Li- ing alcohols and halide ions, which comprise a large group of environmental pollutants [2]. censee MDPI, Basel, Switzerland. This Due to its broad substrate specificity, LinB has a potential for applications in biosensing article is an open access article distributed and biodegradation of environmental pollutants [3]. under the terms and conditions of the The catalytic cycle of LinB is similar to all haloalkane dehalogenases (HLDs) and Creative Commons Attribution (CC BY) was described earlier [4]. The cleavage of the carbon–halogen bond is the fastest step in license (https://creativecommons.org/ catalytic cycle, while the hydrolysis of the alkyl-enzyme intermediate is rate-limiting step licenses/by/4.0/). Catalysts 2021, 11, 5. https://dx.doi.org/10.3390/catal11010005 https://www.mdpi.com/journal/catalysts Int. J. Mol. Sci. 2021, 22, x FOR PEER REVIEW 2 of 16 Structure of LinB is typical for α/β-hydrolase proteins. It consists of conserved α/β core domain and upper α-helical cap domain, which is sequentially and structurally var- ied among dehalogenases [5]. The core domain (residues 3–132, 214–296) consists of a cen- tral twisted eight-stranded β-pleated sheet, flanked by two on one side and four on the other side α-helices. The cap domain (residues 133–213) is formed by five α-helices (Figure 1). LinB belongs to the HLD-II subfamily [6]. The catalytic nucleophile Asp108 is situ- ated at the “nucleophile elbow” between β5 strand and α3 helix, base His272 is located in Catalysts 2021, 11, 5 β8-α11 loop and catalytic acid lies after strand β6. Halide stabilizing residues Asn38 and2 of 15 Trp109 are located on the loops between β3-α1 and β5-α3, respectively [5,7]. The active site cavity of the enzyme is one of the largest among structurally characterized dehalo- 3 genasesin the (276 kinetic A ). Due mechanism to the large [4]. Theactive cap site domain cavity is LinB flexible, prefers allowing to utilize for thelong-chain fast exchange and of bulkyproducts substrates and [8]. substrates The deeply between buried the cavity active of sitethe andenzyme the bulkis connected solvent [with4]. surround- ing solventStructure by tunnels of LinB identified is typical by fortheα Ca/βver-hydrolase program proteins. [9] (Figure It consists 1): p1 (main of conserved tunnel) α/β and p2core (slot, domain [10]). and The upper main αtunnel-helical branches cap domain, into two which tunnels is sequentially with one asymmetric and structurally open- varied ing: p1aamong or upper dehalogenases tunnel (residues [5]. The Gln146, core domain Asp147, (residues Gly176 3–132, and Leu177) 214–296) and consists p1b or oflower a central tunneltwisted (Gln146, eight-stranded Leu177, Ala247,β-pleated Ala271 sheet, and His272) flanked [10,11]. by two on one side and four on the other side α-helices. The cap domain (residues 133–213) is formed by five α-helices (Figure1). Figure 1. Overall structure of the wild type LinB dehalogenase (PDB ID 1cv2, [5]). The upper, lower Figuretunnels 1. Overall and structure slot are shown of the wild as magenta, type LinB cyan dehalogenase and orange (PDB spheres, ID 1cv2, respectively. [5]). The Theupper, catalytic lower triad tunnelsresidues and slot (Asp108, are shown Glu132 as andmagenta, His272) cyan are and shown orange as black spheres, spheres. respectively. The studied The mutations catalytic aretriad shown residuesas red (Asp108, sticks. Glu132 and His272) are shown as black spheres. The studied mutations are shown as red sticks. LinB belongs to the HLD-II subfamily [6]. The catalytic nucleophile Asp108 is situated atArchitecture, the “nucleophile chemical elbow” properties between andβ dyna5 strandmics and of theα3 access helix, basetunnels His272 significantly is located in affectβ enzyme8-α11 loop catalysis and catalytic since they acid have lies aftera majo strandr influenceβ6. Halide on exchange stabilizing rates residues of substrates Asn38 and and productsTrp109 are [10–14]. located In on addition, the loops tunnels between maβ3-y αalso1 and affectβ5- αother3, respectively enzyme properties, [5,7]. The activesuch site as substratecavity of specificity the enzyme and is timing one of thestages largest of the among reactions structurally [15,16]. characterizedTherefore, several dehalogenases vari- 3 ants of(276 LinB A ).have Due been to the designed large active to explore site cavity structure-function LinB prefers relati to utilizeonships long-chain of the enzyme and bulky and optimizesubstrates its [ 8properties]. The deeply for biotechnological buried cavity of applications the enzyme [12]. is connected It has been with experimen- surrounding tally solventdemonstrated by tunnels that identifiedsubstitution by of the Leu1 Caver77 located program in [ 9the] (Figure mouth1): of p1 the (main main tunnel) tunnel and can modifyp2 (slot, the [10 ]).activity The main and tunnelspecificity branches of LinB into enzyme two tunnels [17]. LinB with oneL177W asymmetric variant with opening: Leu177p1a replaced or upper by tunnel bulky (residues Trp shows Gln146, a dramatic Asp147, Gly176decrease and in Leu177)the activity and p1bof the or lowerenzyme tunnel towards(Gln146, the best Leu177, substrate Ala247, 1,2-dibromoethane Ala271 and His272) [17]. [10 Moreover,,11]. the kinetic analysis shows that the introductionArchitecture, of chemicalthe Trp at properties the tunnel andopening dynamics changes of the accessmechanism tunnels of bromide significantly affect enzyme catalysis since they have a major influence on exchange rates of substrates and products [10–14]. In addition, tunnels may also affect other enzyme properties, such as substrate specificity and timing stages of the reactions [15,16]. Therefore, several variants of LinB have been designed to explore structure-function relationships of the enzyme and optimize its properties for biotechnological applications [12]. It has been experimentally demonstrated that substitution of Leu177 located in the mouth of the main tunnel can modify the activity and specificity of LinB enzyme [17].
Details
-
File Typepdf
-
Upload Time-
-
Content LanguagesEnglish
-
Upload UserAnonymous/Not logged-in
-
File Pages15 Page
-
File Size-