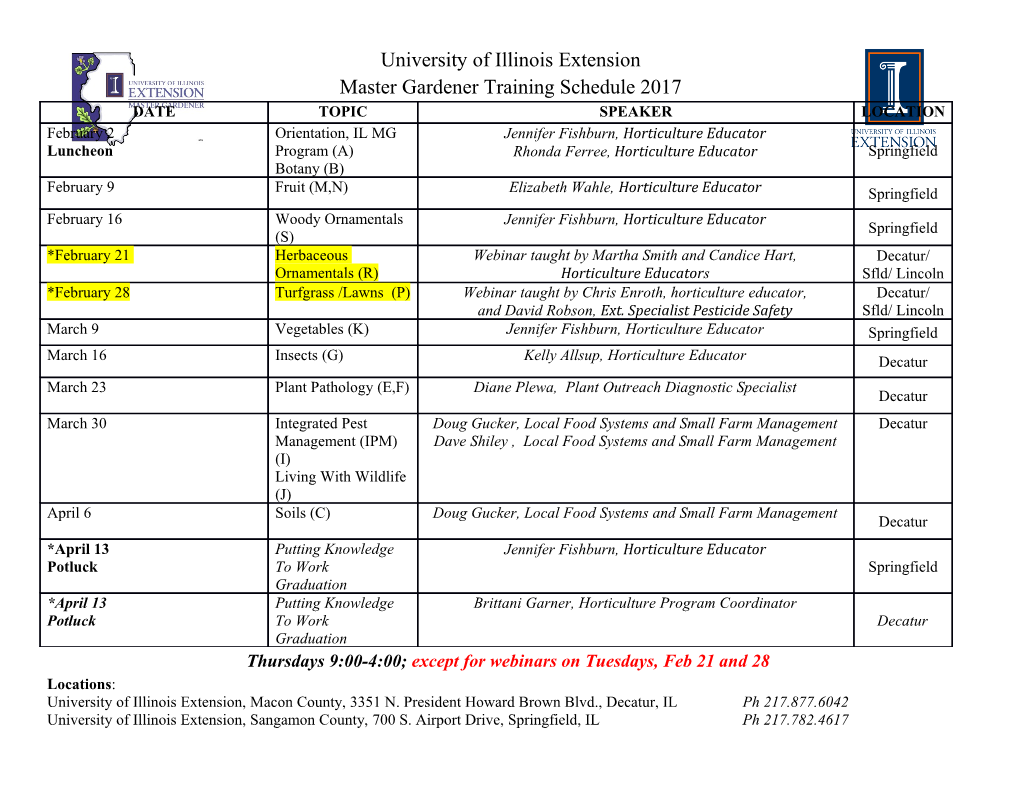
NOVEMBER 2019 S C H L U T O W 3327 Modulational Stability of Nonlinear Saturated Gravity Waves MARK SCHLUTOW Institut fur€ Mathematik, Freie Universitat€ Berlin, Berlin, Germany (Manuscript received 15 March 2019, in final form 13 July 2019) ABSTRACT Stationary gravity waves, such as mountain lee waves, are effectively described by Grimshaw’s dissipative modulation equations even in high altitudes where they become nonlinear due to their large amplitudes. In this theoretical study, a wave-Reynolds number is introduced to characterize general solutions to these modulation equations. This nondimensional number relates the vertical linear group velocity with wave- number, pressure scale height, and kinematic molecular/eddy viscosity. It is demonstrated by analytic and numerical methods that Lindzen-type waves in the saturation region, that is, where the wave-Reynolds number is of order unity, destabilize by transient perturbations. It is proposed that this mechanism may be a generator for secondary waves due to direct wave–mean-flow interaction. By assumption, the primary waves are exactly such that altitudinal amplitude growth and viscous damping are balanced and by that the am- plitude is maximized. Implications of these results on the relation between mean-flow acceleration and wave breaking heights are discussed. 1. Introduction dynamic instabilities that act on the small scale comparable to the wavelength. For instance, Klostermeyer (1991) Atmospheric gravity waves generated in the lee of showed that all inviscid nonlinear Boussinesq waves are mountains extend over scales across which the back- prone to parametric instabilities. The waves do not im- ground may change significantly. The wave field can mediately disappear by the small-scale instabilities, rather persist throughout the layers from the troposphere to the perturbations grow comparably slowly such that the the deep atmosphere, the mesosphere and beyond waves persist in their overall structure over several more (Fritts et al. 2016, 2018). On this range background wavelengths. However, turbulence is produced. Lindzen temperature and therefore stratification and back- (1970) modeled the effect of turbulence on the wave by ground density may undergo several orders of magni- harmonic damping with a constant kinematic eddy vis- tude in variation. Also, dynamic viscosity and thermal cosity. The eddy viscosity is exactly such that it saturates conductivity cannot be considered constant on such a the wave, meaning that viscous damping and anelastic domain (Pitteway and Hines 1963; Zhou 1995). amplification are balanced (Lindzen 1981; Fritts 1984; Two predominant regimes for the waves can be identi- Dunkerton 1989; Becker 2012). Pitteway and Hines (1963) fied: the homosphere and the heterosphere (Nicolet 1954). referred to this instance as amplitude-balanced wave. These two are separated by the turbopause, usually Above the turbopause molecular viscosity takes over. somewhere in the mesopause region. Below the turbo- It is usually modeled by a constant dynamic viscosity. In pause molecular viscosity is negligible. Hence, if diffusion combination with the thinning background density, the occurs, it is caused by turbulence. Due to the missing kinematic viscosity increases exponentially with height damping and the thinning background air, mountain waves resulting in a rapid decrease in amplitude. amplify exponentially when extending upward. This phe- In the process of becoming saturated the amplitude nomenon is also called anelastic amplification. becomes considerably large such that the waves cannot At certain heights the amplitudes cannot grow any be considered linear. Pioneering work on the mathe- further due to limiting processes. Those may be static or matical description of nonlinear gravity waves was ac- complished by Grimshaw (1972, 1974). This paper aims Corresponding author: Mark Schlutow, mark.schlutow@fu- to extend Lindzen’s linear wave saturation theory with berlin.de the aid of Grimshaw’s nonlinear wave description. DOI: 10.1175/JAS-D-19-0065.1 Ó 2019 American Meteorological Society. For information regarding reuse of this content and general copyright information, consult the AMS Copyright Policy (www.ametsoc.org/PUBSReuseLicenses). Unauthenticated | Downloaded 09/27/21 06:58 AM UTC 3328 JOURNAL OF THE ATMOSPHERIC SCIENCES VOLUME 76 y 2. How the modulation equations solve the pffiffiffiffiffiffiffiffir [ Fr 5 O(«1/2), (3b) compressible Navier–Stokes equations—A brief gLr review r y L r r r [ 5 «21 The nonlinear governing equations for our in- m Re O( ), (3c) r vestigations are the two-dimensional dissipative Grimshaw’s c m modulation equations being first introduced by Grimshaw p r [ 5 k Pr O(1), (3d) (1974). Before presenting them, we want to give a brief re- r view in this section how they solve the dimensionless r k m compressible, Reynolds-averaged Navier–Stokes equa- where r, pr, r, and r represent the reference density, tions (NSE) asymptotically. Detailed derivations can be pressure, thermal conductivity, and dynamic viscosity, also found in Achatz et al. (2010) and Schlutow et al. respectively. The constant k 5 2/7 is the ratio of the ideal (2017). Length and time are nondimensionalized via gas constant R to the specific heat capacity at constant pressure cp for diatomic gases. Equations (3) provide a 1 1 y distinguished limit for multiple-scale asymptotic analy- (x^, z^, ^t) 5 x*, z*, r t* , (1) Lr Lr Lr sis. Introducing compressed coordinates for the hori- zontal, vertical, and time axis, where Lr ’ 1...10 km denotes the reference wavelength ^ ^ ^ (hence the subscript r) and yr is the reference velocity. (x, z, t) 5 («x, «z, «t), (4) Note that variables labeled with an asterisk denote di- mensional quantities throughout the paper. To separate separates the slowly varying background from the fast the hydrostatic background from the flow field associ- oscillating wave fields. Under the assumption of stable ated with the wave the first ingredient necessary is a stratification to the leading order, the hydrostatic small scale separation parameter background is determined by the vertical temperature profile T(z). Two dimensionless background variables, « 5 Lr/Hu 1, (2) which vary on the large scale, will appear in the final modulation equations, the Brunt–Väisälä frequency or where Hu ’ 10...100 km is the potential temperature stratification measure N and the background density r. scale height. This choice for the scale separation pa- They have to be calculated from the temperature by rameter was introduced by Achatz et al. (2010). solving The authors considered inviscid flows. To take viscous damping into account, we need to compare inviscid and 1 dT N2 5 1 1 , (5) viscous terms. The (eddy) viscosity is not a constant T dz throughout the atmosphere and among others depends on temperature. Midgley and Liemohn (1966, their 1 dr 1 dT 1 52 1 , (6) Fig. 9) gave a realistic vertical profile of the effective r dz T dz k kinematic viscosity combining eddy and molecular ef- fects. Hodges (1969) computed the eddy diffusion by which originate from the hydrostatic assumption in combination with the ideal gas equation of state. gravity waves near the mesopause to be Kr(eddy) ’ 2 106...7 cm2 s 1, which compares well with Midgley and With the given scale separation of background and Liemohn (1966) and Lindzen (1981). In the scaling re- wave field, we can formulate the scaled two-dimensional gime of Achatz et al. (2010) as well as Schlutow et al. compressible NSE, (2017) these values correspond to Reynolds num- bers of Re ’ 10...100 when the Mach number is Ma ’ Dv 1 =^ 2 52« =^ 2 2 1 «L=^2 1 ... ^ p Nbez (Nb p N pez) v , 0.1...0.01. These numbers are also supported by a review Dt of Fritts (1984). (7a) Taking these arguments into account, a realistic flow Db 1 dN ^ regime in terms of Mach, Froude, Reynolds, and Prandtl 1 Nw 52« N2 1 wb 1 «L=2b 1 ... , (7b) D^t N dz number most suitable for internal gravity waves in the middle/upper atmosphere region is then found by ^ 1 dr = Á v 52« N2 1 w 1 ... , (7c) assuming r dz y pffiffiffiffiffiffiffiffiffiffiffiffiffiffiffiffiffiffiffiffiffiffiffiffir [ Ma 5 O(«), (3a) in terms of the wave-field variables, velocity v, buoyancy 2 k r ^ T (1 )pr/ r Nb, and kinematic pressure p, where = 5 (›/›x^, ›/›z^) Unauthenticated | Downloaded 09/27/21 06:58 AM UTC NOVEMBER 2019 S C H L U T O W 3329 and ez the unit vector pointing in the vertical direction; The modulation equations govern the evolution of ^ D/Dt denotes the material derivative, and L is the total vertical wavenumber kz, wave action density ra,and kinematic viscosity. The dots represent higher-order mean-flow horizontal wind u.Equations(9a)–(9c) are terms that we do not give explicitly but must not be closed by neglected. The interested reader finds these terms in j j2 5 2 1 2 Schlutow et al. [2017, their (2.19)]. k kx kz , (9d) Wentzel–Kramers–Brillouin (WKB) theory provides Nk us with a multiple-scale method to solve the scaled NSE v 5 x 1 k u, (9e) asymptotically by the spectral ansatz jkj x f « ^ ^ ^ « 5 1 i (x,z,t)/ 1 0 Nkxkz U(x, z, t; ) U0,0(x, z, t) [U0,1(x, z, t)e c.c. ] v 52 , (9f) jkj3 1 h.h. 1 O(«), (8) where k, v, and v0 represent the wavenumber vector, where c.c. stands for the complex conjugate and h.h. for extrinsic frequency, and vertical linear group velocity, higher harmonics. The vector U 5 (v, b, p)T contains the respectively. Note that primes denote derivative with prognostic variables and f is the wave’s phase. respect to the vertical wavenumber throughout this pa- We want to give a remark at this point. By construc- per. Extrinsic frequency is defined by the sum of intrinsic tion, the WKB ansatz is a nonlinear approach.
Details
-
File Typepdf
-
Upload Time-
-
Content LanguagesEnglish
-
Upload UserAnonymous/Not logged-in
-
File Pages10 Page
-
File Size-