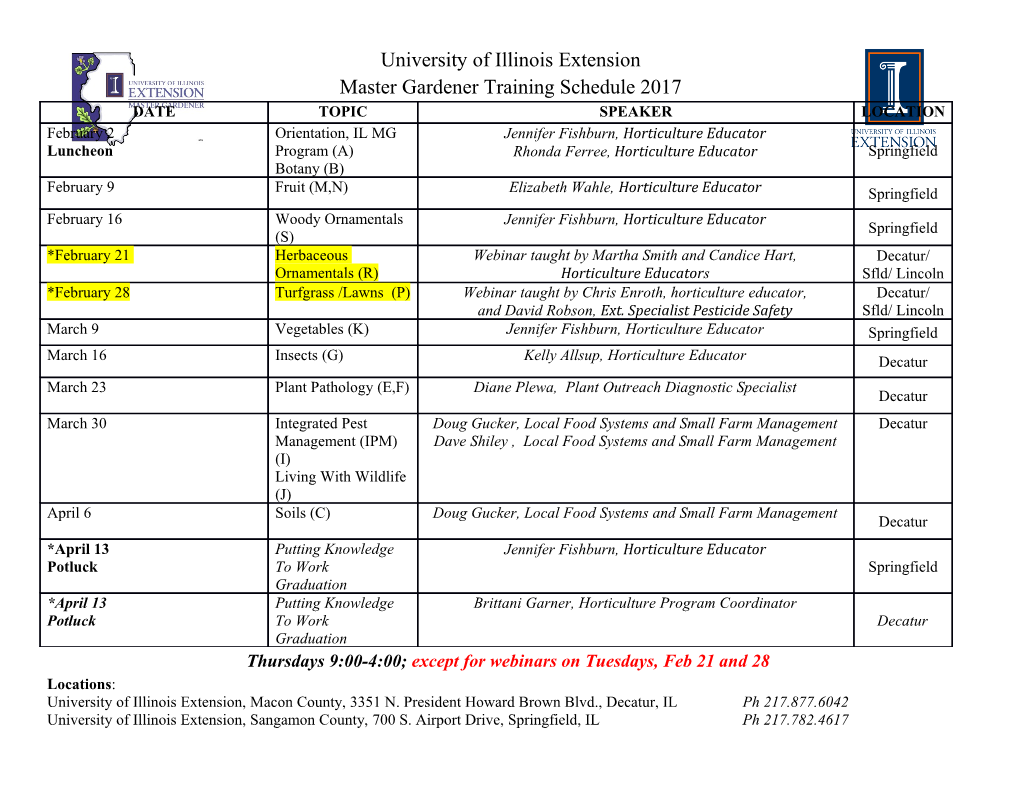
On-Site Opportunities for GW Detection Christoph Reinhardt 26.05.2021 Overview Contribute to existing/future detectors Development of technologies (LIGO, Virgo, KAGRA, ET, NEMO, …) (cooling concepts, …) On-site GW detector/s Provide infrastructure (based on axion experiments, (e.g., Cryoplatform at HERA North) new dedicated detector) 1 Cryogenic Test Masses (in collaboration with R. Schnabel‘s group, UHH) • Increasing strain sensitivity requires cryogenic cooling of suspended test masses to lower mirror and suspension thermal noise or to suppress thermal distortion ET-LF design (test mass @ 10 - 20 K) [1] NEMO design (test mass @ 123 - 150 K) [2] Test mass suspended for seismic isolation: [1] Abernathy, Matt, et al. "Einstein gravitational wave Telescope conceptual design study." (2011) [2] Ackley, K., et al. "Neutron Star Extreme Matter Observatory: A kilohertz-band gravitational-wave detector in the global network." Publications of the Astronomical Society of Australia 37 (2020) 2 Cryogenic Test Masses (in collaboration with R. Schnabel‘s group, UHH) Heat conduction via suspension fibers Radiative cooling via Gas cooling via black body radiation helium exchange gas Monolithically connected fibers with simultaneously high thermal High-emissivity coating on mirror barrel Gas particles transfer heat from test conductivity and low mechanical loss mass to frame → Limited heat transfer for thin fibers → Limited effectiveness at ~ 20 K → Cooling at cost of additional noise from impinging helium atoms 3 Gas Cooling of Test Masses (in collaboration with R. Schnabel‘s group, UHH) Relation between cooling power and corresponding displacement noise (gaskinetic theory, free molecular flow): Displacement Noise ∝ 푃1/2푚−1α−1/2 cooling power mirror mass energy accommodation coefficient Heat Transfer Displacement Noise Just for model comparison [Reinhardt et al. `2021] 4 Gas Cooling Applied to NEMO (in collaboration with R. Schnabel‘s group, UHH) Target: neutron star merger • Tidal effects during the inspiral (푓 ≳ 500 Hz) • Post-merger signal (푓 > 1 kHz) Characteristics • Circulating power of 4.5 MW • Test masses radiatively cooled to 123 K / 150 K (to prevent thermal distortion) Up to ~1022 Hz1/2 Potential benefit of gas cooling • Higher sensitivity by increasing circulating power [Ackley et al. `2020] 푓 × Strain 5 Gas Cooling Applied to NEMO (in collaboration with R. Schnabel‘s group, UHH) Target: neutron star merger • Tidal effects during the inspiral (푓 ≳ 500 Hz) • Post-merger signal (푓 > 1 kHz) Characteristics • Circulating power of 4.5 MW • Test masses radiatively cooled to 123 K / 150 K (to prevent thermal distortion) Potential benefit of gas cooling • Higher sensitivity by increasing circulating power [Ackley et al. `2020] 6 Characterization of heat transfer (in collaboration with R. Schnabel‘s group, UHH) • Cryogenic test setup to measure efficiency of helium gas cooling, depending on energy accommodation coefficient → investigate surface enhancing coating Silicon Cylinder Cryostat at UHH 7 Cryoplatform at HERA North • Distribution system of liquid helium (at ~ 4.5 K) supplying multiple experimental setups (e.g., MADMAX) • Availability of high magnetic fields (~ 5 T) • Located 30 m underground at HERA North (next to ALPS IIc) • HERA North hall dimensions: height = 16 m, area = 1222 m² PhotographPhotograph of HERA North 8 Possible on-site opportunities • Resonant masses • SRF cavities • MADMAX • Levitated sensors • BabyIAXO (Image courtesy of Francesco Muia) 9 Resonant-Mass Detectors Concept Resonant amplification of mechanical strain from incident GW to detectable levels 3rd Generation Detectors (Length ~ 3 m) Detector Material Mass (kg) Frequency (Hz) Bath T (K) ALLEGRO 4.2 7 푄m~10 AURIGA 4.5 Al5056 ~2300 ~900 EXPLORER 2.6 NAUTILUS 2.5 http://www.auriga.lnl.infn.it/auriga/detector/overview.html NIOBE Nb 1500 ~700 5.0 [Ronga et al. `2006] Strain Sensitivity (1/Hz1/2) Bandwidth (Hz) Measured @5 K ~10-20 ~100 Hz First longitudindal mode Expected @100 mK ~10-21 ~150 Hz 푇 Thermal Noise ∝ 푚푄m 10 Resonant-Mass Detectors Concept Resonant amplification of mechanical strain from incident GW to detectable levels 3rd Generation Detectors (Length ~ 3 m) Detector Material Mass (kg) Frequency (Hz) Bath T (K) ALLEGRO 4.2 AURIGA 4.5 Al5056 ~2300 ~900 EXPLORER 2.6 NAUTILUS 2.5 [Tobar et al. `1995] NIOBE Nb 1500 ~700 5.0 [Ronga et al. `2006] Strain Sensitivity (1/Hz1/2) Bandwidth (Hz) Measured @5 K ~10-20 ~100 Hz Expected @100 mK ~10-21 ~150 Hz 푇 Thermal Noise ∝ 푚푄m Source 11 Resonant-Mass Detectors https://www.minigrail.nl/ Spherical Detectors • MiniGRAIL, Mario Schenberg GW Antenna • Spherical design with quadrupole mode Material Mass (kg) Frequency (Hz) Bath T (K) CuAl 1400 ~2900 20 mK 1/2 65 cm Strain Sensitivity (1/Hz ) Bandwidth (Hz) 7 푄m~10 Measured @5 K ~10-20 ~100 Hz Predicted @50 mK ~10-22 ~400 Hz [Oliveira et al. `2016] [Gottardi et al. `2007] Prospects • Six spherical detectors with masses from 200 to 1200 kg Targeted Sources → strain sensitivity of 10-22 Hz1/2 from 3 to 10 kHz • non-axisymmetric instabilities in rotating single [ Aguiar 2011 ] and binary neutron stars • Dual sphere with optical cavity readout • small black-hole or neutron-star mergers (finesse: 1e6, input power 12 W, material: Mo or Be, mass: ~10 t, mech. Q factor: 1e8, bath T: 1K) → Strain sensitivity 10-23 Hz1/2 for 1 to 3 kHz ~1 m (comparable to aLIGO) [Cerdonio 2001] 12 Superconducting RF Cavity Concept (MAGO) • GW interacting with cavity walls transfers ~ 50 cm (~ 5kg) energy between coupled cavity modes with electromagnetic frequencies 푓1, 푓2 • Sensitive to GWs at 푓1 − 푓2 • Spherical shape maximizes electromagnetic Q 10 푄em ≳ 10 factor for given surface resistance Coupled Nb Cavity (Proposed) Tune 푓1 −푓2 From 4 to 20 kHz Cell Radius 0.2 m MAGO (former collaboration between CERN & INFN) Wall Thickness 5 mm [Ballantini et al. `2003] Mass 44 kg El.-mag. Resonance 1 GHz El.-mag. Q Factor 8.5×1011 Sensitivity -21 1/2 Mech. Resonance 2 kHz • 6×10 Hz from 1.8 to 2.2 kHz (near mech. resonance, quantum limited amplifier) • 10-22 Hz1/2 over sub-hertz bandwidth Mech. Q Factor 106 13 Superconducting RF Cavity Concept (MAGO) • GW interacting with cavity walls transfers energy between coupled cavity modes with electromagnetic frequencies 푓1, 푓2 • Sensitive to GWs at 푓1 − 푓2 • Spherical shape maximizes electromagnetic Q factor for given surface resistance Coupled Nb Cavity (Proposed) Cell Radius 0.2 m Wall Thickness 5 mm Mass 44 kg El.-mag. Resonance 1 GHz 10 W @ 1.5 to 1.8 K El.-mag. Q Factor 8.5×1011 6 Mech. Resonance 2 kHz 푄m~10 Mech. Q Factor 106 (limited by LHe) [Ballantini et al. 2005] 14 Superconducting RF Cavity Mechanical Resonance in Superfluid Helium Resonant Frequency Conversion Concept GW excites mech. resonance in superfluid helium → Upconversion of red-detuned pump to cavity resonance 11 푄He~10 @10mK 8 푄He~10 @20mK [S. A. R. Ellis' Slides] Prototype in dilution refrigerator: Prototype in dilution refrigerator: a = 11 cm, L = 3 m, M = 16 kg, a = 1.8 cm, L = 4 cm, M = 6 g, Mech. Resonance: 1 kHz Mech. Resonance: 10 kHz El.-mag. Resonance: 휔푖 ~ GHz Tunability: 훿휔 ~ MHz Strain Sensitivity Magnetic Field: 퐵 = 0.2 T Strain Sensitivity (Predicted) 0 10-18 to 10-26 Hz1/2 -23 1/2 Q Factor: 푄 = 1012 4×10 Hz (10 kHz to 10 MHz) (@1 kHz, tunable by 50 % via pressurization of He) Volume: 푉 = 1 m3 Temperature: 푇 = 1.4 K [Singh et al. 2017] 15 MADMAX & Magnetic Conversion 10 – 100 GHz [Beurthey et al. `2020 ] [Egge et al. `2020] Strain Sensitivity for single photon detector @ 40 GHz SPD ℎCGMB = 2.4 × 10−25 ∝ 휖−1/2Γ1/4퐵−1퐿−1퐴−1/2 [Ringwald et al. `2021] 푐 sens SP detection Dark count efficiency rate 16 BabyIAXO & Magnetic Conversion 70 cm [Abeln et al. 2020] Strain Sensitivity for single photon detector @ 40 GHz SPD ℎCGMB = 3.5 × 10−25 ∝ 휖−1/2Γ1/4퐵−1퐿−1퐴−1/2 [Ringwald et al. `2021] 푐 sens SP detection Dark count efficiency rate 17 Axion Experiments & Magnetic Conversion [Ejlli et al. `2019] Required • Development of broadband µ-wave SPDs at 40 GHz (exists: TWPAs at 6-7 GHz, 3-4 GHz bandwidth, nearly quantum-limited) • Dilution refrigerator(s) to operate SPDs at 100 mK (to suppress thermal noise) • Integration with MADMAX/BabyIAXO MADMAX BabyIAXO (µ-Wave Detector) 40 GHz (Image courtesy of Francesco Muia) 18 −11 Levitated Sensor Detector Limited by gas damping (푝~10 mbar) −10 13 Silica Disc: 푚~10 kg, 푄eff~10 Concept 15 µm • Passing GW causes a harmonic force to displace an optically-trapped disk inside an optical cavity from its equilibrium position • Resonance tunable from 10 to 300 kHz via laser trap 75 µm Strain Sensitivity Ultra-low noise SiN membrane resonators instead of dielectric disks? [Reinhardt et al. `2016] [Beccari et al. `2021] Parameters: Structural Damping: 푓~100 kHz Strain Noise ∝ 푇/푄푚 −12 푚~10 kg 4 300 K → 30 mK 푄푚 increase by 10 ? [Arvanitaki et al. `2013] 푄 ~109 [Aggarwal et al. `2020] 300K (low T, optical trapping) 19 Conclusion • On-site technology development: gas cooling of test masses with dedicated test setup • DESY infrastructure may enable (e.g., at Cryoplatform, HERA North) • Realization of full-scale prototypes • GW detectors based on resonant masses, SRF cavities, … • Equipping MADMAX and BabyIAXO with single photon detectors as a first step toward detection of CGMB • Other options to be explored, e.g., nanomechanical membranes as levitated sensors 20.
Details
-
File Typepdf
-
Upload Time-
-
Content LanguagesEnglish
-
Upload UserAnonymous/Not logged-in
-
File Pages21 Page
-
File Size-