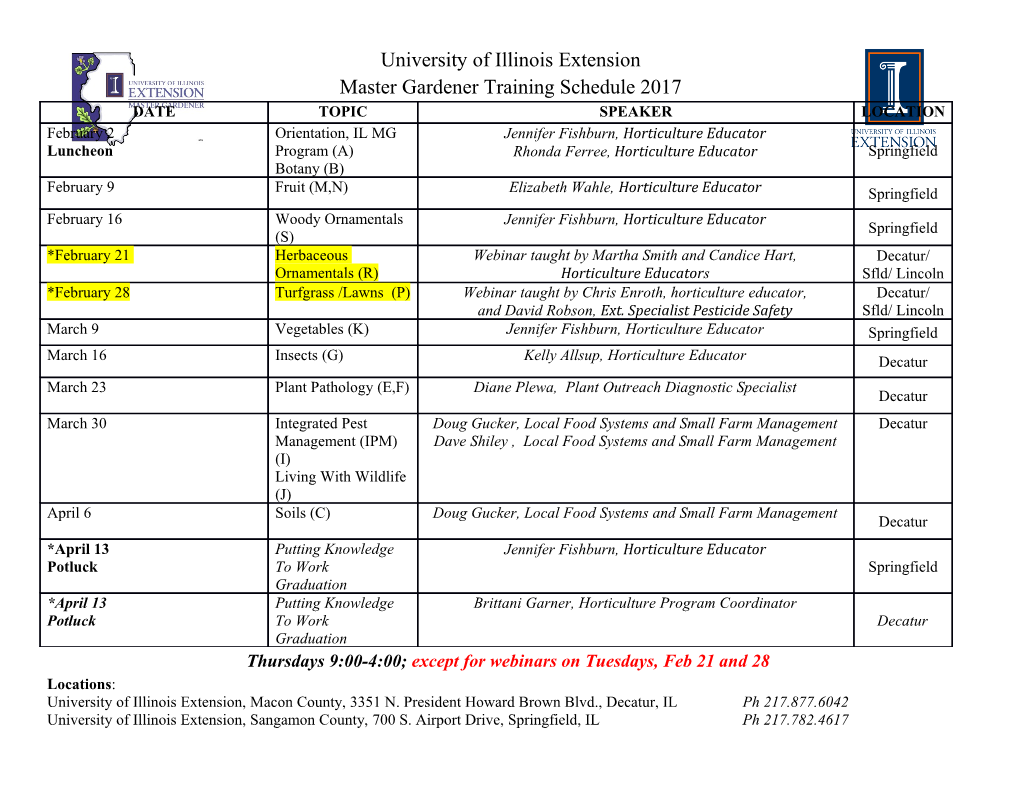
<p> 1 The presynaptic ribbon maintains vesicle populations at the <a href="/tags/Hair_cell/" rel="tag">hair cell</a> afferent fiber </p><p>2 synapse </p><p>3 Lars Becker1, Michael E. Schnee1, Mamiko Niwa1*, Willy Sun2, Stephan Maxeiner3**, </p><p>4 Sara Talaei1, Bechara Kachar2, Mark A. Rutherford4, Anthony J. Ricci1,3 </p><p>5 1 Department of Otolaryngology Stanford University, United States, 2 National Institute </p><p>6 of Deafness and Communicative Disorders, United States, 3 Molecular and Cellular </p><p>7 Physiology, Stanford University, United States, 4 Department of Otolaryngology, </p><p>8 Washington University in St. Louis, United States, </p><p>9 *Present address: Department of Otolaryngology Johns Hopkins University, United </p><p>10 States </p><p>11 ** Present address: Department of Neuroanatomy, Institute for Anatomy and Cell </p><p>12 Biology, Medical School Saarland University, Germany </p><p>13 </p><p>14 </p><p>15 </p><p>16 </p><p>17 </p><p>18 </p><p>19 </p><p>1 </p><p>20 Abstract: The ribbon is the structural hallmark of cochlear inner hair cell (IHC) afferent </p><p>21 synapses, yet its role in information transfer to spiral ganglion <a href="/tags/Neuron/" rel="tag">neurons</a> (SGNs) remains </p><p>22 unclear. We investigated the ribbon’s contribution to IHC synapse formation and </p><p>23 function using KO mice lacking RIBEYE. Despite loss of the entire ribbon structure, </p><p>24 synapses retained their spatiotemporal development and KO mice had a mild hearing </p><p>25 deficit. IHCs of KO had fewer synaptic vesicles and reduced exocytosis in response to </p><p>26 brief depolarization; high stimulus level rescued exocytosis in KO. SGNs exhibited a </p><p>27 lack of sustained excitatory postsynaptic currents (EPSCs). We observed larger </p><p>28 postsynaptic glutamate receptor plaques, potentially in compensation for the reduced </p><p>29 EPSC rate in KO. Surprisingly, large amplitude EPSCs were maintained in KO, while a </p><p>30 small population of low amplitude slower EPSCs was increased in number. The ribbon </p><p>31 facilitates signal transduction at physiological stimulus levels by retaining a larger </p><p>32 residency pool of synaptic vesicles. </p><p>33 </p><p>34 </p><p>35 Key words: auditory hair cell, synaptic transmission, RIBEYE, glutamate receptor, </p><p>36 multivesicular release </p><p>37 </p><p>38 </p><p>39 </p><p>40 </p><p>2 </p><p>41 Introduction </p><p>42 The synaptic ribbon is an electron dense structure associated with presynaptic active </p><p>43 zones at sensory synapses in the retina, lateral line and inner ear organs. RIBEYE, the </p><p>44 major protein associated with the ribbon (Zanazzi and Matthews 2009), consists of a </p><p>45 unique amino-terminal A domain that forms the ribbon scaffold and a carboxy-terminal B </p><p>46 domain with lysophosphatidic acid acyltransferase activity which is almost identical to </p><p>47 CtBP2, a transcriptional co-repressor (Schmitz, Konigstorfer et al. 2000). The ribbon is </p><p>48 anchored to the presynaptic membrane by bassoon protein in mammalian IHCs </p><p>49 (Khimich, Nouvian et al. 2005) as well as retinal photoreceptors and bipolar cells (dick </p><p>50 2001). Although it is comprised predominantly of RIBEYE (Zenisek, Horst et al. 2004), </p><p>51 the ribbon complex may associate with over 30 different synaptic proteins (Uthaiah and </p><p>52 Hudspeth 2010, Kantardzhieva, Peppi et al. 2012). </p><p>53 IHCs respond to sound with graded receptor potentials that drive rapid and precise </p><p>54 synaptic release, encoding intensity and timing information (Fuchs 2005, Matthews and </p><p>55 Fuchs 2010). The ribbon is thought to sustain continuous encoding by tethering a pool </p><p>56 of vesicles that replenishes the local pool of docked and primed vesicles upon </p><p>57 depletion. Other functions ascribed to the ribbon include: regulating vesicle trafficking </p><p>58 from the cytoplasm to the <a href="/tags/Active_zone/" rel="tag">active zone</a>, serving as a vesicle trap to bind freely diffusing </p><p>59 vesicles, a location for vesicle formation, a physical barrier for Ca2+ ensuring a high </p><p>60 local Ca2+ signal, and as a primary structural component of the synapse anchoring Ca2+ </p><p>61 channels close to synaptic vesicle release sites (Parsons 1994, Frank, Rutherford et al. </p><p>62 2010, Graydon, Cho et al. 2011, Kantardzhieva, Peppi et al. 2012). </p><p>3 </p><p>63 Postsynaptic EPSCs at ribbon synapses range in amplitude by up to 20X. The smallest </p><p>64 and largest EPSCs have similar kinetics, which together suggested the existence of a </p><p>65 mechanism to synchronize the release of multiple quanta (multivesicular release, MVR; </p><p>66 (Glowatzki and Fuchs 2002, Li, Keen et al. 2009, Schnee, Castellano-Munoz et al. </p><p>67 2013, Rudolph, Tsai et al. 2015). By holding vesicles and voltage-gated Ca2+ channels </p><p>68 at the active zone the ribbon may support MVR, occurring through various mechanisms </p><p>69 including: i) coordinated fusion of multiple single vesicles, ii) release of large pre-fused </p><p>70 vesicles, iii) rapid sequential fusion in which the 1st fusion event triggers fusion of </p><p>71 additional vesicles, or some combination of these mechanisms (Matthews and Fuchs </p><p>72 2010). </p><p>73 Previous attempts to study ribbon function used genetic disruption of the anchoring </p><p>74 protein Bassoon or acute destruction of the ribbon by fluorophore assisted light </p><p>75 inactivation (FALI). These manipulations resulted in smaller vesicle pool size, calcium </p><p>76 channel mislocalization, increased auditory thresholds, asynchrony in neural responses </p><p>77 and reductions in both tonic and phasic responses (Snellman, Mehta et al. 2011, Jing, </p><p>78 Rutherford et al. 2013). However, neither of those manipulations were selective for the </p><p>79 ribbon and so might be manifestations of accessory protein denaturing. </p><p>80 Recently, manipulation of the gene encoding CtBP2/RIBEYE allowed for deletion of </p><p>81 RIBEYE by removing the A-domain of ctbp2 to eliminate ribbons in mice, enabling a </p><p>82 more precise examination of ribbon function. This mouse will be referred to as KO and </p><p>83 the littermate controls as WT throughout the manuscript. Elimination of ribbons in the </p><p>84 retina reduced phasic and tonic transmission and rendered release sites sensitive to the </p><p>85 slow calcium buffer EGTA (Maxeiner, Luo et al. 2016). Here we present analysis of </p><p>4 </p><p>86 cochlear synaptic anatomy, hair cell and afferent fiber physiology and hearing function </p><p>87 in the RIBEYE knockout mouse. Results are distinct from those in retina and from a </p><p>88 RIBEYE deletion in zebrafish (Lv, Stewart et al. 2016) , suggesting a more subtle </p><p>89 function for the ribbon than previously assumed. </p><p>90 Results: </p><p>91 IHC-Spiral Ganglia <a href="/tags/Neuron/" rel="tag">Neuron</a> (SGN) synapses are formed and maintained despite </p><p>92 absence of the ribbon: The inner ear structure of the KO was assessed first for the </p><p>93 presence of synaptic ribbons and synapses (Maxeiner, Luo et al. 2016). Validating loss </p><p>94 of hair cell ribbons was accomplished using immunohistochemistry for the RIBEYE B-</p><p>95 domain (CtBP2). Anti-CtBP2 labelled the ribbons in wildtype (WT) but not in the KO </p><p>96 animals (postnatal day 21, P21)(Fig. 1A). Nuclear CtBP2 was still observed in both WT </p><p>97 and KO (not shown) because the KO is specific to the RIBEYE A-domain. The nuclear </p><p>98 staining served as a usful control for antibody function. </p><p>99 Using antibodies to Homer for postsynaptic labeling and Bassoon for presynaptic </p><p>100 labeling (Fig. 1B,C) we quantified the number of puncta and whether puncta were </p><p>101 juxtaposed as synaptic pairs (Fig. 1I,J). Bassoon and Homer puncta were similar in </p><p>102 number between WT and KO (Bassoon: 40 ± 15 in WT, vs. 43 ± 12 in KO, p = 0.76; </p><p>103 Homer: 17 ± 3 for both WT and KO; WT: n = 20 mice, KO: n = 19 mice, p = 0.64). The </p><p>104 number of paired Homer and Bassoon puncta (Fig. 1D) were similarly comparable: </p><p>105 14 ± 2 (n = 20) for WT and 13 ± 2 (n = 19, p = 0.18) for KO IHCs, suggesting that </p><p>106 synapses were formed and maintained in comparable numbers regardless of the </p><p>107 presence of ribbons. The higher number of Bassoon puncta likely reflects its localization </p><p>108 at efferent synapses. Figure 1- Supplemental Fig. 1 presents a lower magnification </p><p>5 </p><p>109 image that illustrates the multiple roles of Bassoon at the auditory periphery. Bassoon is </p><p>110 a major component of the presynaptic elements at the auditory periphery including the </p><p>111 efferent fibers and the hair cell afferent synapses. Thus it is not surprising that paired </p><p>112 Bassoon puncta are much less than total puncta because Bassoon serves multiple </p><p>113 synapses. Similarly, when interpreting Bassoon KO data it is not surprising that its </p><p>114 phenotype may be more dramatic than simply the RIBEYE effect alone due to number </p><p>115 of synapses involved and schaffolding function of Bassoon that leads to structural </p><p>116 changes at the synapse. </p><p>117 RIBEYE KO in zebrafish revealed a non osmiophilic structure resembling a ribbon that </p><p>118 maintained organization of synaptic vesicles in the absence of the RIBEYE protein in </p><p>119 hair cells of the lateral line neuromasts (Lv, Stewart et al. 2016). We examined whether </p><p>120 such a structure existed in the mammalian cochlea in the IHCs of the KO using </p><p>121 transmission electron microscopy (TEM) and electron-tomography. Representative </p><p>122 examples of conventional TEM (Fig. 1E, 70 nm sections) and tomographic </p><p>123 reconstructions (Fig. 1F,G at 10 nm tomographic sections) are presented. The KO </p><p>124 presynaptic synapses consistently showed a small osmiophilic density in contact with </p><p>125 the pre-synaptic membrane at the center of the synapse that resembled the arciform </p><p>126 density seen at ribbon synapses in the retina. This density may be the Bassoon </p><p>127 enriched linker between the membrane and the ribbon. Interestingly, this density is not </p><p>128 observed in the Bassoon KO mouse (Frank et al., 2010). Tomographic reconstructions, </p><p>129 (Fig. 1G, right) clearly show the presynaptic density, which is smaller than the ribbon </p><p>130 and is present also at ribbon synapses in WT IHCs (Fig. 1G, left). We found no spatial </p><p>131 organization of vesicles near active zones in KO IHCs, suggestive of the ‘ghost’ ribbons </p><p>6 </p><p>132 observed in the zebrafish lateral-line hair cells (Lv, Stewart et al. 2016). Thus, in the KO </p><p>133 mouse, IHCs lack synaptic ribbons and have no obvious compensatory structures. We </p><p>134 surmise that the differences observed in the zebrafish model are a function of the KO </p><p>135 being incomplete or there being a compensation mechanism in zebrafish, not found in </p><p>136 mammal. </p><p>137 KO IHCs may upregulate a similar protein to replace RIBEYE loss. CtBP1 is a likely </p><p>138 candidate because it is similar to the RIBEYE-B domain, is expressed in hair cells and </p><p>139 localizes to the synaptic region (Kantardzhieva, Liberman et al. 2013). Antibody labeling </p><p>140 (Fig. 1H,K,L) demonstrates the presence of CtBP1 in both WT and KO animals which </p><p>141 overlapped with Homer in WT. A reduction in puncta number was identified in the KO </p><p>142 animal due to loss of localization at the afferent synapses (Fig. 1K,L). Thus, the CtBP1 </p><p>143 localization at the synapse requires the presence of RIBEYE, and CtBP1 does not </p><p>144 substitute for RIBEYE in the KO animal. </p><p>145 Synaptic vesicles are normal in size but fewer in number: Vesicle diameters </p><p>146 (distance between the outer edges of the bilayer in each vesicle) were measured in the </p><p>147 reconstructed tomograms. We measured in both X and Y directions with no differences </p><p>148 observed. In the WT the average vesicle diameter was 33.6 ± 3 nm (n = 21) and in the </p><p>149 KO it was 33.8 ± 3 nm (n = 21). </p><p>150 Synaptic vesicles were conspicuously fewer in number the KO. Vesicles were counted </p><p>151 in volumes around the center of the reconstructed synapses (Fig. 1G), where one </p><p>152 boundary was the presynaptic membrane. The volumes had XY dimensions of 453 ± 6 </p><p>153 nm for WT (n = 3) and 569 ± 117 nm for KO (n = 11), and Z dimensions of 88 ± 3 and </p><p>154 95 ± 10 nm for WT and KO, respectively. Total vesicle numbers were 20 ± 2 for WT and </p><p>7 </p><p>155 7 ± 3 for KO, significantly greater for WT compared with KO synapses (p < 0.001, Welch </p><p>156 correction for unequal variance). Our TEM data is qualitatively similar to that reported in </p><p>157 the companion paper and supports the conclusion that ribbons are not present in the </p><p>158 KO. </p><p>159 Auditory nerve activity is reduced in absence of synaptic ribbons: Previous </p><p>160 investigation of ribbonless synapses in the KO mouse focused on the retina and did not </p><p>161 assess function at the systems level (Maxeiner et al., 2016). To determine how hearing </p><p>162 was affected, we measured ABRs and distortion product otoacoustic emissions </p><p>163 (DPOAEs). DPOAEs are a direct measure of outer hair cell function and cochlear </p><p>164 amplification. DPOAEs are not expected to be affected by absence of RIBEYE and </p><p>165 serve as a control for developmental acquisition of mature cochlear mechanics. DPOAE </p><p>166 threshold measurements were not different across frequencies from 4 - 46 kHz (Fig. </p><p>167 2A). Data are presented in decibels (dB) as referenced to sound pressure level (SPL) </p><p>168 with lower values indicating enhanced sensitivity in the mid-cochlea. Normal DPOAEs </p><p>169 indicate that cochlear amplification was not effected mechanically by the loss of outer </p><p>170 hair cell ribbons, suggesting that sound-driven activation of IHCs was intact, and that </p><p>171 any differences in auditory nerve activity should result from changes at the IHC afferent </p><p>172 synapses. </p><p>173 Wave 1 of the ABR is a direct measurement of the synchronized firing of SGNs at </p><p>174 sound onset, triggered by synaptic output from cochlear IHCs. The subsequent waves </p><p>175 of the ABR reflect the ensuing activation of brainstem nuclei in the ascending auditory </p><p>176 pathway (Zheng, Henderson et al. 1999). ABR thresholds were elevated by </p><p>177 approximately 10 dB at all probe frequencies in KO mice (Fig. 2B), except for 48 kHz </p><p>8 </p><p>178 where hearing threholds are already relatively high. The variance in our control data </p><p>179 ranged from 8-12dB depending on frequency; this dictated the sample size needed to </p><p>180 detect a 5% change as statistically different. ABR threshold was identified as the </p><p>181 stimulus intensity required to evoke a voltage response 5x the RMS noise floor for the </p><p>182 measurement. The companion paper found a similar elevation in threshold though it did </p><p>183 not reach statistical significance. The difference is likely due to sampling size where we </p><p>184 powered our sample sized based on variance found in control measurements. The ABR </p><p>185 waveform (Fig. 2C: 23 kHz, 60 dB) shows a large reduction in wave 1 amplitude with no </p><p>186 effect on the subsequent peaks. This was surprising and suggests considerable </p><p>187 plasticity in central processing of data translated from cochlea periphery. KO mice had </p><p>188 smaller wave 1 peak amplitudes, for all sound levels that evoked a response (Fig. 2D). </p><p>189 When wave 1 growth functions were plotted relative to the maximal response amplitude </p><p>190 within genotype, the amplitude versus level function for KO scaled up to overlap with </p><p>191 WT (Fig. 2E). Considering wave 1 amplitude as a fraction of the maximal amplitude, the </p><p>192 overlap of the normalized wave 1 growth curves suggested that loss of maximum output </p><p>193 could produce an elevation of threshold in the ABR measurement that does not </p><p>194 necessarily reflect a sound detection limit or a change in sensitivity of the auditory </p><p>195 system. Indeed, normal ABR amplitudes beyond wave 1 indicate compensation in the </p><p>196 cochlear nucleus. Future work should determine if KO mice have increased behavioral </p><p>197 thresholds. </p><p>198 In mice lacking Bassoon function, where IHCs lacked most of their membrane-anchored </p><p>199 ribbons, there was an increase in wave 1 latency (Khimich, Nouvian et al. 2005) and in </p><p>200 first-spike latency in recordings of SGNs in vivo (Buran, Strenzke et al. 2010). ABR </p><p>9 </p><p>201 wave 1 latencies were not different between WT and KO at any stimulus intensity (Fig. </p><p>202 2F), suggesting a milder phenotype in the KO as compared to Bassoon. </p><p>203 As the ABR measurements are averaged responses of 260 stimulus repetitions, a </p><p>204 possible explanation for the reduced ABR wave 1 amplitude in KO is that the response </p><p>205 reduces over time. If the ribbon is providing a means of maintaining vesicles near the </p><p>206 synapse, the concomitant loss of vesicles at ribbonless synapses might reduce the </p><p>207 ongoing response to repetitive stimulation. Comparison of ABR amplitudes where the </p><p>208 number of repeats was varied from 260 to 1000 showed no difference in amplitudes, nor </p><p>209 did repeated measurements meant to evoke depletion.Thus, rundown alone is unlikely </p><p>210 to account for the decrease in ABR wave 1. </p><p>211 Characterization of molecular synaptic components indicates an increase in </p><p>212 postsynaptic glutamate receptor distribution: To begin identifying cellular </p><p>213 mechanisms that might underlie the measured reduction in ABR wave 1 amplitude we </p><p>214 investigated the distribution of presynaptic Ca2+ channels and postsynaptic glutamate </p><p>215 receptors as indicators of functional synapses. Figure 3 presents immunohistochemical </p><p>216 localizations of presynaptic ribbons with anti-CtBP2, presynaptic voltage-gated Ca2+ </p><p>217 channels with anti-CaV1.3, and postsynaptic AMPA-type glutamate receptors with anti-</p><p>218 GluA3 in P35 mice organ of corti. There was no difference in the number of puncta </p><p>219 between WT and KO for CaV1.3 (20 ± 6, n = 9 vs. 18 ± 5, n = 13, p = 0.51) or GluA3 (15 </p><p>220 ± 3, n = 9 vs. 15 ± 2, n = 13, p = 0.43) (Fig. 4A,B). There was also no difference in the </p><p>221 number of paired synaptic puncta between genotypes, with values of 14 ± 2 (n = 9) for </p><p>222 WT and 13 ± 2 (n = 13) for KO (p = 0.13). Thus, the presence of the ribbon did not </p><p>223 influence the number of apparently functional synapses per IHC. </p><p>10 </p><p>224 Careful inspection of GluA3 immunoreactivity showed that the glutamate receptor </p><p>225 puncta were larger on average in the KO (Fig. 4D). Puncta volumes were measured for </p><p>226 presynaptic markers CaV1.3 and Bassoon as well as postsynaptic markers Homer and </p><p>227 GluA3 (Fig. 4C). Like GluA3, Homer puncta were significantly larger (KS-test, </p><p>228 p ≤ 0.0001) on average in the KO (Fig. 4D). The volumes of CaV1.3 and Bassoon </p><p>229 distributions were very similar among genotypes however the volume of Bassoon was </p><p>230 significantly higher in KO. Most WT and KO synapses exhibited the typical elongated </p><p>231 stripe-like appearance of CaV1.3 puncta. However, we noted a tendency for KO </p><p>232 synapses to have CaV1.3 clusters that were fragmented into smaller puncta (Fig. 3C, </p><p>233 arrow; see also Companion Paper). These anatomical data support the hypothesis that </p><p>234 the ribbon is not a major regulator of synapse formation or stability; however, it does </p><p>235 perhaps suggest that postsynaptic receptors are sensitive to activity and the increased </p><p>236 plaque size might reflect a compensation for reduced activity. </p><p>237 Basolateral IHC properties: We compared the properties of the IHC voltage-gated </p><p>238 Ca2+ current (Fig. 5), which drives exocytosis. We first blocked outward K+ currents </p><p>239 using Cs+ and tetraethylammonium (TEA) in the internal solution to isolate Ca2+ currents </p><p>240 in WT and KO IHCs (Fig. 5A,B). Current-voltage plots showed no difference in voltage </p><p>241 of half-activation (V1/2) or maximum current amplitude (Fig.5C-E). Half activation </p><p>242 voltages were -34 ± 0.3 mV (n = 18, WT) and -33.4 ± 0.3 mV (n = 26, KO) for animals </p><p>243 P10-P13. The companion paper found small changes in voltage dependent properties </p><p>244 of the calcium channels at older ages and in higher external calcium; differences that </p><p>245 might suggest compensatory effects of ribbon loss or later specializations being ribbon </p><p>246 dependent. Examples of current elicited with K+ as the major internal cation and without </p><p>11 </p><p>247 TEA are presented in Fig. 5F,G. Recordings were made at P20-24. No differences were </p><p>248 observed in maximal current or in V1/2 (Fig. 5H). Zero-current potentials monitored in </p><p>249 current-clamp mode (Fig. 5I) were not different, with means of -63 ± 10 mV (n = 5, WT) </p><p>250 and -65 ± 8 mV (n = 8, KO). </p><p>251 Release is slower and smaller for naturalistic stimuli in absence of the ribbon: We </p><p>252 measured presynaptic exocytosis with the two-sine wave method for tracking vesicular </p><p>253 fusion by monitoring real time changes in cellular membrane capacitance (Cm) from </p><p>254 IHCs (Santos-Sacchi 2004, Schnee, Castellano-Munoz et al. 2011). The two-sine </p><p>255 method allows continuous monitoring of Cm during the depolarizing stimulus as </p><p>256 compared to a before and after monitoring of capacitance changes, which is particularly </p><p>257 helpful during smaller, longer depolarizations (Schnee, Santos-Sacchi et al. 2011). </p><p>258 Typically, small depolarizations lead to relatively slow, linear increases in Cm during </p><p>259 depolarization. In contrast, larger depolarizations induce an abrupt departure from </p><p>260 linearity termed the ‘superlinear’ response (Schnee et al. 2011). Both types of </p><p>261 responses were present in recordings from IHCs of WT and KO mice alike (Fig. 6A,B). </p><p>262 From Cm measurements, we estimated the sizes of synaptic vesicle pools, their rates of </p><p>263 release and the Ca2+-dependence of release. In response to strong depolarizations, we </p><p>264 found no difference in maximal release between genotypes for either the linear or </p><p>265 superlinear release components (Fig. 6C). The linear component was 52 ± 24 fF (n = </p><p>266 15, WT) compared to 42 ± 22 fF (n = 15, KO); and the superlinear component of release </p><p>267 was 223 ± 79 fF (n = 17, WT) compared to 253 ± 116 fF (n = 17, KO). Likewise, no </p><p>268 differences in release rates (Fig. 6D) were observed for the linear component: 101 ± 72 </p><p>269 fF/s (n = 15, WT) compared to 75 ± 50 fF/s (n = 14, KO) or for the superlinear </p><p>12 </p><p>270 component: 256 ± 164 fF/s (n = 17, WT) and 253 ± 117 fF/s (n = 18, KO). In addition, </p><p>271 the Ca2+-dependence of release for the linear component was not different (0.9 ± 0.03 </p><p>272 fF/pC (n = 37, WT) as compared to 0.93 ± 0.03 fF/pC (n = 34, KO). The voltage at which </p><p>273 the superlinear component of release first appears was also not different between mice </p><p>274 (Fig. 6E). Fitting the data with a simple Gaussian function gave values of -40 ± 9 mV for </p><p>275 WT and -41 ± 3 mV for KO for the center values. However the width of the functions </p><p>276 were different, with values of 7.6 ± 2 for WT compared to 14.3 ± 3 for KO (values p< </p><p>277 0.002 level). Thus, although the variance in release properties increased for the KO </p><p>278 animal, there were no major differences in the ability of the synapses to respond to </p><p>279 strong stimuli. </p><p>280 In contrast to the comparable maximal responses, smaller depolarizations revealed a </p><p>281 reduction in release at early time points in the KO. The difference observed was </p><p>282 masked at longer time points or by stronger depolarization (Fig. 6F-J). Comparing </p><p>283 capacitance changes at short intermediate and longer time points in response to smaller </p><p>284 depolarizations showed that the KO consistently had reduced release as compared to </p><p>285 the WT data. Fitting the data in Fig. 6G,H with a simple exponential demonstrated that </p><p>286 although both genotyes reached the same values of release with strong depolarizations </p><p>287 the relationships varied in two ways. For the shortest time point release started at -40 </p><p>288 mV as compared to -45 mV with all other variables remaining constant. Given that the </p><p>289 numbers for release are quite small and variable, conclusions should be conservative, </p><p>290 but support the argument that fewer vesicles are available for release at this early time </p><p>291 point and recruitment is minimal. For the longer time point Fig. 6H, the voltage-</p><p>292 dependence (Cm/Vm) varied with the KO, 7.3 ± 3.6 for WT as compared to 12.7 ± 5.8 </p><p>13 </p><p>293 for KO. F-tests show these fits to be different at the p < 0.002 level. Longer time points </p><p>294 as depicted in Fig. 6I,J show no difference between groups. In addition, for smaller </p><p>295 depolarizations the delay to release onset was greater in the KO IHCs, consistent with a </p><p>296 reduction in vesicles immediately available for release (Fig 6K). For example, </p><p>297 depolarizations to -44 mV resulted in significantly shorter latency in WT (102 ± 98 ms, n </p><p>298 = 16) compared to KO (204 ± 166 ms, n = 17, p = 0.039). Stronger depolarizations (> -</p><p>299 39 mV) resulted in no difference in release latency. The time constants of endocytosis </p><p>300 were not significantly different (6.0 ± 4s, n = 13, WT and 7 ± 5 s, n = 13, KO). Together </p><p>301 these data suggest that fewer vesicles were available for release at ribbonless </p><p>302 synapses, but that with stronger depolarizations or longer time courses of depolarization </p><p>303 the recruitment of additional vesicles masked this immediate difference in release </p><p>304 latency and amplitude. </p><p>305 The ribbon may be a barrier to Ca2+ diffusion, creating a restricted space for sharp Ca2+ </p><p>306 nanodomains beneath the ribbon (Roberts 1994, Graydon, Cho et al. 2011). </p><p>307 Intracellular Ca2+ buffering can alter release properties depending on the relative </p><p>308 distribution of Ca2+ channels with release sites. In retina the KO increases buffering </p><p>309 efficacy (Maxeiner et al. 2016), suggesting looser coupling between Ca2+ channels and </p><p>310 release sites. To investigate the effects of ribbon absence on stimulus-secretion </p><p>311 coupling in p11 to p13 IHCs, we compared between genotypes the effects on release </p><p>312 properties of changing concentration of the slow Ca2+ buffer EGTA (Fig. 7). Examples of </p><p>313 release obtained with either 0.1 or 5 mM EGTA are presented in Fig. 7A (control </p><p>314 experiments all use 1 mM EGTA). Although increasing buffer concentration reduced </p><p>315 release and lowering buffer increased release, the relative changes were comparable </p><p>14 </p><p>316 between WT and KO mice for release measured at 1 sec (Fig. 7B). Data previously </p><p>317 demonstrated that increasing Ca2+ buffering increased the time to superlinear release </p><p>318 (Schnee, Santos-Sacchi et al. 2011). Fig. 7C demonstrates that this effect is retained in </p><p>319 the KO and that there are no major differences between WT and KO modulation by </p><p>320 Ca2+ buffering. These results contrast with findings from retinal bipolar cells and in the </p><p>321 zebrafish KO (Maxeiner, Luo et al. 2016); see Discussion). Similarly, release rates </p><p>322 measured for steps to -45 mV were not significantly different for the initial release </p><p>323 components (Fig. 7D). We further investigated the first component of release, typically </p><p>324 thought to represent vesicles in early release pools, not requiring trafficking. Comparing </p><p>325 release at two voltages again showed that the buffer greatly altered release properties </p><p>326 but not in a differential way between genotypes. However, it should be noted that the </p><p>327 difference in early release observed in Fig. 6 with 1 mM EGTA was lost with 0.1 mM </p><p>328 EGTA. Thus, it is possible that a sensitivity exists that is below a clear detection limit for </p><p>329 our capacitance measurements. </p><p>330 EPSCs are more heterogeneous in size and kinetics in the absence of the ribbon: </p><p>331 Cm measurements are a powerful tool for investigating whole-cell exocytosis but they </p><p>332 lack sensitivity (1 fF reflects fusion of ~20 vesicles), they reflect information from all </p><p>333 synapses and they can be quite variable. To obtain a more refined view of activity from </p><p>334 individual synapses we recorded postsynaptically, from the afferent fiber bouton. Hair </p><p>335 cells bathed in artificial perilymph lack the standing inward current through </p><p>336 mechanoelectric transduction channels in hair bundles, causing the resting membrane </p><p>337 potential to be relatively hyperpolarized (Farris, Wells et al. 2006, Johnson, Kennedy et </p><p>338 al. 2012). To depolarize hair cells and increase the rate of vesicle fusion, we applied a </p><p>15 </p><p>339 high potassium (40 mM K+) solution to depolarize the hair cell. K+ application to the hair </p><p>340 cell in current-clamp mode depolarized hair cells by an average of 32 ± 5 mV (n = 8; </p><p>341 Fig. 8A). There was no difference in depolarization between genotype thus allowing </p><p>342 conclusions regarding postsynaptic responses to be ascribed to presynaptic effects. </p><p>343 Afferent boutons of type I auditory nerve fibers were recorded ex vivo at ages of P17-</p><p>344 P21. Due to the approach vector of the recording pipette, recordings were made from </p><p>345 boutons located on the side of the IHC facing the ganglion (modiolar face) rather than </p><p>346 the pillar cell side. The start of the high K+ response was defined when the mean EPSC </p><p>347 rate exceeded the rate in normal external solution by 2 standard deviations. Examples </p><p>348 of postsynaptic responses to K+ applications are provided in Figure 8B-D. Two types of </p><p>349 responses were observed, sustained responses (Fig. 8B,C) and transient responses </p><p>350 (Fig. 8D). WT responses were mainly of the sustained type (7 of 8) while the KO </p><p>351 responses had a smaller proportion of sustained responses (7 of 11). EPSC rates are </p><p>352 plotted for transient (Fig. 8E) and sustained responses (Fig. 8F) for both WT and KO. </p><p>353 EPSC rates in 40 mM K+ perilymph were quantified over one second windows at 100 </p><p>354 ms intervals and the means are displayed as thicker lines (Fig. 8F). The peak release </p><p>355 rates varied considerably across fiber populations (0-120 s-1) and there was a tendency </p><p>356 for the KO neurons to reach a lower peak EPSC rate on average: 57 ± 30 s-1, (n = 11, </p><p>357 WT) compared with 44 ± 31 s-1, (n = 18, KO) (Fig. 8G). </p><p>358 The time to peak EPSC rate was less variable than absolute rates (Fig. 8H). The KO </p><p>359 had one outlier that steadily increased throughout the K+ application, removal of this </p><p>360 point from the statistical analysis resulted in the KO reaching peak EPSC rate sooner (9 </p><p>361 ± 10 sec, n = 10) than WT (21 ± 12 sec, n = 9; p = 0.037). Observing the sustained </p><p>16 </p><p>362 release group shows that both WT and KO reduced EPSC rate over time but that the </p><p>363 KO reduced more completely than WT (Fig. 8G). 8/11 KO reduced to less than 5% of </p><p>364 peak as compared to 3/8 WT. Integrating the rates over time (Fig. 8I) confirmed an </p><p>365 increase in total EPSCs in WT (1.8 ± 1.1 x 103) compared to KO (0.81 ± 0.66 x 103, p = </p><p>366 0.021). </p><p>367 EPSC amplitude distributions were generated for each recorded fiber. Frequency </p><p>368 histograms of EPSC amplitudes could be fit with either a double Gaussian function or a </p><p>369 Gaussian and gamma function where the smallest peak was always fit by a Gaussian </p><p>370 function (e.g. Fig. 8J,K). The area under the smaller peak was calculated and plotted as </p><p>371 a percentage of the total in Fig. 8L, where the KO showed a statistically larger (p = </p><p>372 0.034) percentage of small events with 5.5 ± 5.6% (n = 12) as compared to 1 ± 0.7% (n </p><p>373 = 7). The increase in number of small events might suggest less coordination in release </p><p>374 because there are fewer vesicles filling release sites. It might also suggest that smaller </p><p>375 responses are visible because of an increased in receptor plaque size as well. Only </p><p>376 fibers with more than 100 EPSCs were included for this analysis. No difference was </p><p>377 found in the proportion of simple events for each unit, where a simple event is described </p><p>378 as having a single rise and decay phase (Fig. 8M). We also compared the median </p><p>379 amplitudes (Fig. 8N). Although there was a tendency for EPSCs in the KO to be greater </p><p>380 in amplitude 212 ± 46 pA (n = 13) compared to 173 ± 36 pA (n = 7), the values were not </p><p>381 statistically different. A difference here would support the argument of a postsynaptic </p><p>382 compensatory increase in glutamate receptor plaque size. The coefficient of variation </p><p>383 (CV) for the amplitude distributions were different on average (p = 0.017; Fig. 8O). The </p><p>384 control values are similar to those obtained by (Li, Keen et al. 2009, Grant, Yi et al. </p><p>17 </p><p>385 2010). Recordings with a larger proportion of smaller events had larger CV. When small </p><p>386 events were removed from the histogram, the CVs were not statistically different </p><p>387 between KO and WT. The postsynaptic responses in KO also had a greater proportion </p><p>388 of EPSCs > 350 pA (Fig. 8J,K, 9D): 1.3 ± 1.3% in WT compared to 8.0 ± 10% for KO </p><p>389 EPSCs (t-test for unequal variances, p = 0.04). The change in amplitude was not </p><p>390 accompanied by a change in kinetics. The increased amplitude might reflect the </p><p>391 increase in postsynaptic glutamate receptor plaque (Fig. 3,4). </p><p>392 Examples of EPSCs are presented in Fig. 9A to show the variation in amplitudes and to </p><p>393 identify a smaller population of EPSCs with slower kinetics (see inset). As the ribbon is </p><p>394 proposed to be involved with multivesicular release (MVR), and the kinetic similarities </p><p>395 between large and small EPSCs are a signature of MVR, we investigated the decay of </p><p>396 EPSCs in auditory nerve fiber recordings from WT and KO animals. Fitting the EPSC </p><p>397 decay with single exponentials provided time constants (Fig. 9B). Calculating the CVs of </p><p>398 EPSC decay time constants (Fig. 9C) revealed more variability in KO but no significant </p><p>399 difference compared to WT. The skewness (Fig. 9F) of the decay time constant </p><p>400 distributions was significantly larger for the KO (0.46 ± 0.2, n = 13) compared to WT </p><p>401 (0.29 ± 0.07, n=7; p = 0.04); the KO mean EPSC decay time was greater than the </p><p>402 median decay time. As a population 0.44% of WT EPSCs were < 100 pA in amplitude </p><p>403 with decay time constants > 1 ms while 1.9% of KO events fell in that category. Plotting </p><p>404 the decay time constant against amplitude for each EPSC in all fibers (Fig. 9D) (WT: n = </p><p>405 7 recordings, 15,564 EPSCs; KO: n = 13 recordings, 17,210 EPSCs) identifies a </p><p>406 population of small slow EPSCs in the KO that are present in greater proportion than in </p><p>407 WT (upper left quadrant). The mean EPSC decay time constants plotted as a function of </p><p>18 </p><p>408 EPSC amplitude for each recording (bin of 10 pA) illustrates that all the KO fibers had a </p><p>409 proportion of EPSCs with slow time courses while the WT have only 2 fibers with these </p><p>410 slower events (Fig. 9E). An example of the smaller, slower EPSC is provided in the </p><p>411 inset of Fig. 9A. The distributions of EPSC durations for all EPSCs < 100 pA (Fig. 9G,H) </p><p>412 demonstrates the shift toward slower longer duration EPSCs in KO fibers (p > 0.001, </p><p>413 using the Kolmogorov-Smirnov test). The cumulative probability functions of EPSC </p><p>414 duration for all fibers by genotype (thick red and blue lines) illustrates the larger </p><p>415 proportion of slow EPSCs in the KO fibers (Fig. 9H). These data may also support the </p><p>416 argument for a larger postsynaptic glutamate receptor plaque, where diffusion of </p><p>417 glutamate, coupled with the lower glutamate concentration might serve to slow the </p><p>418 decay kinetics. </p><p>419 Discussion: </p><p>420 The KO mouse provides an unprecedented opportunity to investigate the functional </p><p>421 relevance of synaptic ribbons in driving information transfer at the primary auditory </p><p>422 synapse. Synapse formation and stability appeared normal based on synapse counts </p><p>423 and protein localization, despite complete absence of the ribbon (Figs. 1, 3-4). No </p><p>424 evidence was found for partial or defective ribbons structures, nor did we detect </p><p>425 compensation by other proteins in the absence of RIBEYE (Fig. 1). Also, cochlear </p><p>426 mechanics appeared normal as assessed by DPOAE (Fig. 2). Thus, this mouse </p><p>427 provides an excellent model to investigate the ribbon’s role in synaptic transmission. </p><p>428 Whole animal function: ABR responses reported a 10 dB threshold shift and about a </p><p>429 50% reduction in ABR amplitude with no significant effect on the shape of the </p><p>430 waveforms or on the delay (Fig. 2). These data agree qualitatively with the companion </p><p>19 </p><p>431 paper data, the lack of significance likely being a function of the sample size. The minor </p><p>432 auditory deficit may not be expected to produce a change in behavioral sound detection </p><p>433 threshold as the ensuing ABR peaks, representing brainstem nuclei, were unaffected. </p><p>434 Alternatively, central compensation might occur that mask peripheral deficits. These </p><p>435 data suggest that the ribbon is not essential for setting hearing threshold, and that its </p><p>436 role in auditory function is more nuanced than for example otoferlin which when absent </p><p>437 or altered leads to deafness with threshold elevations of more than 100 dB (Roux, </p><p>438 Safieddine et al. 2006, Pangrsic, Lasarow et al. 2010). Further work is needed to better </p><p>439 understand ribbon function at the systems level. Additional testing to better investigate </p><p>440 auditory processing such as sound localization experiments may provide additional </p><p>441 insight into how the ribbon might serve systems level function. </p><p>442 Synapse formation: Synapse formation is surprisingly normal with appropriate number </p><p>443 of synapses and synaptic markers present (Figs. 1,4). Little change in Ca2+ channel </p><p>444 distribution or biophysics were observed in the KO (Fig. 5) relative to Bassoon </p><p>445 disruption, suggesting that Ca2+ channel distribution or regulation does not depend </p><p>446 critically on the ribbon (Frank, Rutherford et al. 2010). Our companion paper identifies </p><p>447 minor changes in Ca2+ channel distribution and voltage sensitivity at later ages; whether </p><p>448 these are a function of ribbon absence or activity dependent modulation remains to be </p><p>449 explored. The lack of effect on calcium channel properties and distributions at early </p><p>450 ages supports the hypothesis of a compensatory mechanism occurring later during </p><p>451 synapse maturation. Given the reports of late development changes in ribbon size and </p><p>452 glutamate receptor plaque sizes, activity driven shaping of the synapses is a reasonable </p><p>453 expectation (Fernandez, Jeffers et al. 2015). Postsynaptically, both Homer and the </p><p>20 </p><p>454 glutamate receptors had larger volumes in the KO animal than in WT (Fig. 4). This </p><p>455 change also may be an indication of activity dependent synaptic shaping where the </p><p>456 reduced activity in the KO animal results in a postsynaptic up regulation of receptors. </p><p>457 The increase in the population of larger amplitude EPSC’s in KO animals further </p><p>458 supports this conclusion (Figs 8I,J, 9D). </p><p>459 Comparison to other systems: In zebrafish a frameshift mutation was used to </p><p>460 eliminate ribeye from ribbon synapses. This work revealed ghost like ribbons where </p><p>461 vesicles were organized about invisible ribbon-like structures (Lv C et al.,2016). Neither </p><p>462 our investigations, nor the companion paper nor the original work investigating the </p><p>463 retina (Maxeiner et al., 2015) found comparable ghosts in this mouse. We would </p><p>464 conclude that either the frame shift approach did not account for all ribeye or there is a </p><p>465 strong compensatory mechanism in zebrafish that is not found or is much weaker in </p><p>466 mammal. We investigated CTBP1 as a potential compensatory molecule but as Fig. 1 </p><p>467 shows there is no labeling near synapses in the KO mouse. The presence of ribbons in </p><p>468 photoreceptors of zebrafish support the idea that there are fundamental differences </p><p>469 between zebrafish and mammalian systems. Additionally, work in zebrafish suggests a </p><p>470 tight reciprocal relationship between calcium channel numbers, calcium current </p><p>471 amplitude and ribbon size (Lv, Stewart et al. 2016). As no changes in calcium channel </p><p>472 distribution or numbers were observed in the KO animal despite the loss of the ribbon, </p><p>473 this relationship does not appear to exist in mammal or is greatly reduced, again </p><p>474 supporting fundamental differences between model organisms. </p><p>475 Mechanism of ABR amplitude reduction: The decrease in ABR amplitude is likely a </p><p>476 function of the reduced frequency of EPSCs leading to fewer action potentials </p><p>21 </p><p>477 generated (Fig. 8F). In addition, the increase in proportion of EPSCs with lower </p><p>478 amplitudes and longer durations (Fig. 9H) is expected to increase the likelihood of spike </p><p>479 generation failure (Rutherford, Chapochnikov et al. 2012). As larger EPSCs are better </p><p>480 phase-locked than smaller EPSCs (Li, Cho et al. 2014) and present works finds a 5-</p><p>481 10% increase in smaller EPSCs one might predict a lower vector strength and reduced </p><p>482 phase locking. Our companion paper in fact does observe these differences in single </p><p>483 unit firing. Similarly the increased population of smaller EPSCs is reminiscent of </p><p>484 observations in young animals when compared to older animals and so might reflect a </p><p>485 developmental delay in the KO animal. Fewer fibers fire together at sound onset, </p><p>486 reducing auditory nerve synchrony and wave 1 amplitude (Fig 2C,D). Measurements of </p><p>487 exocytosis from IHCs in response to small depolarizations, clearly showed a reduction </p><p>488 in release and an increase in time to release in the KO (Fig. 6E,F). This suggests a </p><p>489 decrease in vesicles available at the synapse for immediate release (supported by TEM </p><p>490 counts). The reduction in available vesicles for release as well as the population of </p><p>491 reduced amplitude EPSCs might also predict a spike timing deficit as described in the </p><p>492 companion paper. However, the more rapid response in WT was masked by stronger </p><p>493 stimulation (Fig. 6F), indicating that vesicle replenishment remained robust despite the </p><p>494 absence of the ribbon-associated synaptic vesicle pool. Given the robust nature of </p><p>495 vesicle trafficking, the primary role for the ribbon maybe in ensuring a population of </p><p>496 vesicles is present for the timing of onset responses, whereas vesicle trafficking can </p><p>497 serve to replenish vesicle populations during sustained release. A similar result was </p><p>498 reported by (Maxeiner, Luo et al., 2016) in retina. </p><p>22 </p><p>499 Postsynaptic recordings suggest that the KO reaches peak release sooner than WT and </p><p>500 to a similar peak rate, implying that vesicle numbers are comparable for immediate </p><p>501 release. In contrast, TEM suggests fewer vesicles available at the synapse and the </p><p>502 small increase in population of small EPSCs also suggests a reduction in filled release </p><p>503 sites. Thus, it is possible that the ribbon acts as a barrier for vesicle interaction with the </p><p>504 release site while simultaneously serving to increase the total vesicles number </p><p>505 available. Interestingly, this idea was first suggested by (Furukawa and Matsuura 1978) </p><p>506 and later supported by (Schnee, Castellano-Munoz et al. 2013) as a possible </p><p>507 mechanism for explaining neural adaptation. However, the companion paper suggests </p><p>508 modifications at the synapse that might also be in accord with the postsynaptic </p><p>509 measurements here as well, i.e. more vesicles at the membrane. If there is an increase </p><p>510 in presynaptic density area and more vesicles per active zone, it might be predicted that </p><p>511 faster immediate release occurs but sustainability might be reduced, EPSC data also </p><p>512 supports this idea. To resolve these possibililities we need a better understanding of the </p><p>513 molecular underpinnings of the active zone and number of available release sites. </p><p>514 Synaptic vesicle replenishment for sustained release: Our data would argue against </p><p>515 a role for the ribbon in vesicle formation and replenishment. Total exocytosis, from both </p><p>516 the linear and superlinear components, was not reduced in the KO, implying a similar </p><p>517 total number of vesicles that could be recruited for release (Figs. 6A,B and 7A). In </p><p>518 contrast to zebrafish lateral line (Lv, Stewart et al. 2016), we measured no change in </p><p>519 vesicle size between KO and WT, indirectly supporting our view that the ribbon is not </p><p>520 regulating or involved in vesicle formation, a result corroborated by the companion </p><p>23 </p><p>521 paper. Thus, no change in vesicle size, or maximal release supports the conclusion that </p><p>522 the ribbon is not involved in vesicle formation. </p><p>523 Ca2+ influx and coupling to exocytosis: The ribbon may act as a barrier to Ca2+ </p><p>524 diffusion, creating a higher level of local [Ca2+] to synchronize vesicle fusion of multiple </p><p>525 vesicles (Graydon, Cho et al. 2011). In this case, the ribbon’s presence would reduce </p><p>526 the sensitivity of exocytosis to exogenous Ca2+ buffers (by elevating concentrations of </p><p>527 Ca2+). Likewise in retina from KO mice, EGTA reduced spontaneous release in the KO </p><p>528 but not in WT (Maxeiner, Luo et al., 2016). In contrast, our data shows that buffering </p><p>529 efficacy was not altered in the KO (Fig. 7), suggesting the ribbon does not play a major </p><p>530 role in regulation of intracellular Ca2+ concentration at IHC active zones. The similar </p><p>531 response between WT and KO might indicate a small effect though at a level below a </p><p>532 clear detection limit for capacitance measurements. It is possible that the geometric </p><p>533 differences and synaptic architecture between retina and cochlea alter the sensitivity to </p><p>534 Ca2+ buffers or that the effect noted in retina is indirect, for example EGTA-AM might </p><p>535 alter the presynaptic cell’s resting potential. Thus, our results are not fundamentally </p><p>536 different from (Maxeiner, Luo et al., 2016), both supporting the idea that the ribbon </p><p>537 serves to consolidate vesicles near to release sites as suggested by (von Gersdorff, </p><p>538 Vardi et al. 1996). </p><p>539 Ribbon and Multiquantal release: The underlying mechanism for multiquantal release </p><p>540 remains an open question but might be driven by simultaneous multiquantal or </p><p>541 multivesicular release (Glowatzki and Fuchs 2002). The majority of EPSCs measured in </p><p>542 the KO remain large and uniformly fast in onset and decay times and the EPSC </p><p>543 amplitude is not reduced suggesting that the ribbon does not play a pivotal role in </p><p>24 </p><p>544 multivesicular release. Thus, mechanisms that involve sequential fusion of vesicles </p><p>545 along the ribbon and then release or rapid sequential vesicle release that piggyback </p><p>546 onto a release site from the ribbon are unlikely to be valid as these should be </p><p>547 dramatically altered by the loss of the ribbon. One remaining possibility is the </p><p>548 synchronous release of vesicles from multiple release sites. Although the ribbon is not </p><p>549 required for this mechanism, it may indirectly modulate this mechanism by influencing </p><p>550 the number of docked vesicles available for release. The increase in number of smaller </p><p>551 slower EPSCs likely arising from fewer docked vesicles available for synchronous </p><p>552 release may support a ribbon role; however, the small relative contribution of this </p><p>553 vesicle pool indicates a minor role. The phenomenon of large EPSC amplitude </p><p>554 variability may still be explained by a univesicular mechanism including a dynamic </p><p>555 fusion pore, which presumably would not require a ribbon (Chapochnikov, Takago et al. </p><p>556 2014). Where identified, the slow footlike response described by (Chapochnikov, </p><p>557 Takago et al. 2014) was not different between genotypes. </p><p>558 Comparison to Bassoon KO: In mice, the effects of KO are subtle compared with </p><p>559 disruption of Bassoon protein. Loss-of-function mutation in Bassoon led to a reduction in </p><p>560 the number of IHC ribbons associated with presynaptic elements, ABR threshold </p><p>561 elevation of 30 dB, longer ABR wave 1 latency and first-spike latency, and fewer Ca2+ </p><p>562 channels at active zones (Khimich, Nouvian et al. 2005, Buran, Strenzke et al. 2010, </p><p>563 Frank, Rutherford et al. 2010, Jing, Rutherford et al. 2013). Thus, the effects of loss of </p><p>564 Bassoon protein (and membrane-anchored ribbons) were greater than loss of RIBEYE </p><p>565 protein, consistent with a more critical role for Bassoon in scaffolding synaptic proteins. </p><p>566 Also given Bassoon’s presence at efferent synaptic endings in the organ of Corti, it is </p><p>25 </p><p>567 not surprising that the functional loss of Bassoon has a greater phenotype than that of </p><p>568 RIBEYE (Supplemental Fig. 1). </p><p>569 Summary: A central tenet for ribbon function is maintaining a large pool of vesicles </p><p>570 near the synapse. The ribbon may serve as a conveyor belt to both maintain and to </p><p>571 traffic vesicles to release sites (Parsons 1994, Nouvian, Beutner et al. 2006). Our data </p><p>572 suggests the ribbon increases the time a vesicle remains in the synaptic region. Robust </p><p>573 vesicle trafficking and replenishment either via recycling or transporting new vesicles to </p><p>574 the synaptic zone provides vesicles to the synaptic zone, but the time vesicles remain </p><p>575 near to release sites may be shortened in the absence of the ribbon serving to maintain </p><p>576 them close to the synapses. A model for this basic idea was proposed (Graydon, Zhang </p><p>577 et al. 2014) that reproduced data from bipolar cell synapses. The addition of a more </p><p>578 robust trafficking mechanism may allow this model to also reproduce hair cell synapses. </p><p>579 The increase in variance, whether measured as CV, skewness or simply standard </p><p>580 deviation in measured parameters such as time to release, release rate and EPSC </p><p>581 amplitude was greater for KO. The increased variance may simply reflect a nonuniform </p><p>582 population of vesicles near the synapse in the absence of the ribbon. Thus, the ribbon </p><p>583 may serve as a biological buffer, maintaining a uniform population of vesicles near </p><p>584 release sites. </p><p>585 Materials and Methods </p><p>586 Animals </p><p>587 Mice of both sexes from a mixed genetic background (C57bl6, 129/Ola) were bred from </p><p>588 heterozygous breeding pairs and genotyped by PCR for the knockout of the A-domain </p><p>589 in Ctbp2 (Maxeiner et al., 2016) while wild-type littermates served as controls. The </p><p>26 </p><p>590 Administrative Panel on Laboratory Animal Care at Stanford University (APLAC </p><p>591 #14345) and Animal Care and use Committee and Washington University in St. Louis </p><p>592 approved all animal procedures. Experiments were performed blinded to genotype. </p><p>593 Auditory measurements: We measured auditory brainstem responses (ABRs), </p><p>594 distortion products of optoacoustic emissions (DPOAEs) in mice of postnatal day 21 as </p><p>595 previously described (Oghalai, 2004). Mice were anesthetized by administering </p><p>596 100 mg/kg ketamine and 10 mg/kg xylazine intraperitoneally and their body temperature </p><p>597 held constantly at 37°C (FHC, DC-temperature controller and heating pad) until they </p><p>598 fully recovered. </p><p>599 Sound stimuli were generated digitally by a self-written software (MATLAB 2009b, </p><p>600 MathWorks), controlling a custom-built acoustic system using a digital-to-analog </p><p>601 converter (National Instruments, NI BNC-2090A), a sound amplifier (TDT, SA1), and </p><p>602 two high frequency speakers (TDT, MF1). The speakers were connected to an ear bar </p><p>603 and calibrated in the ear canal prior to each experiment using a probe-tip microphone </p><p>604 (microphone type 4182, NEXUS conditioning amplifier, Brüel and Kjær). </p><p>605 Auditory Brainstem Responses (ABRs) were recorded by placing three needle </p><p>606 electrodes subcutaneously at the vertex, below the left ear, and a ground electrode </p><p>607 close to the tail. The signals were amplified 10,000 times using a biological amplifier </p><p>608 (Warner Instruments, DP-311) digitized at 10 kHz, and digitally band-pass filtered from </p><p>609 300 to 3,000 Hz. The stimulus for eliciting the ABR was a 5 ms sine wave tone pip with </p><p>610 cos2 envelope rise and fall times of 0.5 ms. The repetition time was 50 ms, and 260 </p><p>611 trials were averaged. </p><p>27 </p><p>612 At each frequency, the peak to peak voltages of ABR signals at stimulus intensities </p><p>613 ranging from 10 to 80 dB sound pressure levels (SPL) were measured in 10 dB steps </p><p>614 and fitted and interpolated to find threshold five standard deviations above the noise </p><p>615 floor. For statistical purposes we defined threshold as 80 dB SPL, if no ABR was </p><p>616 detected. </p><p>617 Distortion Products of Otoacoustic Emission (DPOAE): For stimulation of DPOAEs </p><p>618 two sine wave tones of equal intensity (l1=l2) and 1-second duration were presented to </p><p>619 the ear. The tones ranged from 20 to 80 dB SPL attenuated in 10 dB increments and </p><p>620 the two frequencies (f2 = 1.2f1) ranged from f2 with 4 to 46 kHz. The acoustic signal was </p><p>621 detected by the microphone in the ear bar was sampled at 200 kHz and the magnitude </p><p>622 of the cubic distortion product (2f1-f2) determined by FFT. Averaging 20 adjacent </p><p>623 frequency bins surrounding the distortion product frequency determined the noise floor. </p><p>624 DPOAE thresholds were reached when the signal was greater than three standard </p><p>625 deviations above the noise floor. </p><p>626 Tissue Preparation, Staining and Confocal Microscopy: For staining of pre- and </p><p>627 postsynaptic markers we used antibodies against Homer (Synaptic Systems, 160003, </p><p>628 1:800 dilution), Bassoon (AbCam, ab82958, 1:500 dilution), CtBP1 (BD-Biosciences, </p><p>629 612042, 1:600 dilution), CtBP2/RIBEYE (Santa Cruz Biotechnology, sc-5966, 1:300 </p><p>630 dilution; BD Transduction Lab, 612044, 1:400), CaV1.3 (Alomone Labs, ACC-005, 1:75), </p><p>631 GluA3 (Santa Cruz Biotechnology, sc-7612, 1:200 dilution). Cochlea of P22 or P35 mice </p><p>632 were isolated in HBSS solution or artificial perilymph, a small hole was introduced at the </p><p>633 apex, cochlea were gently perfused through round and oval windows with freshly </p><p>634 prepared 4 % (w/v) PFA (Electron Microscopy Sciences) in PBS until the fixative </p><p>28 </p><p>635 became visible at the apex. Organs were incubated in the same fixative solution for 30 </p><p>636 min at room temperature. Whole mount organs of Corti were micro dissected, the </p><p>637 tectorial membrane removed, the tissue permeabilized for 30 min in 0.5 % (v/v) </p><p>638 Triton X-100/PBS, blocked for 2 h in 4 % (w/v) BSA/PBS at room temperature. Staining </p><p>639 with all primary antibodies was performed overnight at 4°C in the same blocking buffer. </p><p>640 For staining with antibodies against GluA2 and CaV1.3 the protocol was slightly varied: </p><p>641 fixation was 20 min at 4°C, followed by decalcification in 10 % EDTA (Sigma) for 2 h, </p><p>642 permeabilization and blocking was carried out in a blocking buffer containing 16% </p><p>643 donkey serum and 0.3 % Triton X-100. After washing all species appropriate secondary </p><p>644 antibodies (Alexa Fluor 488, 546 or 555, 647: 1:600 dilution in 4 % (w/v) BSA/PBS) </p><p>645 were applied for 1 h at room temperature. After washing, the organs of Corti were </p><p>646 mounted hair bundles facing upward in ProLong Gold© Antifade (Life Technologies). </p><p>647 Samples were imaged using confocal microscopy (LSM700 or LSM880, Zeiss) on 1.4 </p><p>648 NA oil immersion objectives for confocal imaging, and 1.2 NA water immersion objective </p><p>649 for Airyscan imaging. The optimal voxel size was adjusted to the green channel </p><p>650 (confocal x/y/z: 100/100/350 nm; Airyscan x/y/z: 33/33/222 nm). RIBEYE WT and KO </p><p>651 samples were prepared in parallel and images were collected with identical settings. </p><p>652 All images were taken in the apical region, between 200-1000 µm from the apex, of the </p><p>653 organ of Corti. </p><p>654 Image analysis </p><p>655 For three-dimensional analysis of synaptic punctum volume, intensity and </p><p>656 colocalization, we used the Imaris software package (Version 8.4, Bitplane). Puncta </p><p>657 were initially detected using the spot algorithm allowing for different spot sizes and </p><p>29 </p><p>658 elongated point spread function in z-direction (initial seed size x-y/z: CtBP1, Ctbp2, </p><p>659 Homer, 0.44/1.13 µm; Bassoon 0.37/1.13 µm; GluA3 0.7/1.4 µm; CaV1.3 0.56/1.4 µm), </p><p>660 followed by co-localizing the spots with a threshold lower than 1. All counts were </p><p>661 normalized to the number of Inner hair cells in a region of interest. </p><p>662 To estimate the size of synaptic puncta we used the surface algorithm in Imaris. For all </p><p>663 stainings, the surface detail was set to 0.15 µm, background subtraction to 0.562 µm, </p><p>664 and touching object size was 0.750 µm (Bassoon puncta were split at 0.36 µm). </p><p>665 TEM and Tomography </p><p>666 Temporal bones of P21 mice (WT: n = 5, KO: n = 5) were isolated in HBSS, a small </p><p>667 hole was introduced at the apex of the cochlea, gently perfused through round and oval </p><p>668 windows with freshly prepared 4 % (w/v) PFA, 2 % (w/v) Glutaraldehyde (Electron </p><p>669 Microscopy Sciences) in a buffer containing (in mM) 135 NaCl, 2.4 KCl, 2.4 CaCl2, 4.8 </p><p>670 MgCl2, 30 HEPES, pH 7.4 until the fixative became visible at the apex. Temporal bones </p><p>671 were soaked for 4 hours in the same buffer and shipped in PBS. Cochleae were </p><p>672 microdissected out from fixed adult mouse temporal bones and slowly equilibrated with </p><p>673 30% glycerol as cryoprotection. Tissues were then plunge frozen in liquid ethane </p><p>674 at -180C using a Leica Biosystems grid plunger. Frozen samples were freeze </p><p>675 substituted using Leica Biosystems AFS in 1.5% uranyl acetate in absolute methanol </p><p>676 at -90C for 2 days and infiltrated with HM20 Lowicryl resin (Electron Microscopy </p><p>677 Sciences) over 2 days at -45C. Polymerization of the resin was carried out under UV </p><p>678 light for 3 days at temperatures between -45C and 0C. Ultrathin sections at 100 nm </p><p>679 were cut using a Leica ultramicrotome and collected onto hexagonal 300-mesh Cu grids </p><p>680 (Electron Microscopy Sciences). </p><p>30 </p><p>681 Samples were imaged using a 200kV JEOL 2100 with a Gatan Orius 832 CCD camera. </p><p>682 Single images were acquired using DigitalMicrograph (Gatan). Tilt series were acquired </p><p>683 using SerialEM (Mastronarde 2005) at 1 tilt increment from -60 to +60 and </p><p>684 tomographic reconstruction of tilt series and segmentation of tomograms was done with </p><p>685 IMOD software suite (Kremer, Mastronarde et al. 1996). Fiji was used to generate </p><p>686 projection images from tomograms and for cropping, rotating and adjusting the </p><p>687 brightness and contrast of images. </p><p>688 Hair cell recordings: </p><p>689 IHCs from the apical coil of the organ of Corti were dissected from p10-13 Ctbp2 </p><p>690 littermates of either sex, placed on a plastic coverslip and secured with two strands of </p><p>691 dental floss. Cells were visualized with a water immersion lens (100x) attached to a </p><p>692 BX51 Olympus upright microscope. Patch-clamp recordings were obtained at room </p><p>693 temperature (21-23 C) in an external solution containing in (mM) NaCl 140, KCl, 5.4, </p><p>694 CaCl2, 2.8, MgCl2 1, 4-(2-hydroxyethyl)-1-piperazineethanesulfonic acid (HEPES) 10, D-</p><p>695 glucose 6, creatine, ascorbate, Na pyruvate 2, pH 7.4 adjusted with NaOH. 100 nM </p><p>696 apamin was included to block the sK K+ channel. Cesium at 3 mM was used externally </p><p>697 to reduce inward leak current. Pipette solution contained (mM) CsCl 90, TEA, </p><p>698 tetraethylammonium chloride 13, 3 NaATP, MgCl2 4, creatine phosphate 5, ethylene </p><p>699 glycol-bis(β-aminoethyl ether)-N,N,N',N'-tetraacetic acid (EGTA), HEPES 10, pH </p><p>700 adjusted with CsOH to 7.2. </p><p>701 Two-Sine Method </p><p>702 Hair cells were voltage-clamped with an Axon Multiclamp 700B (Axon Instruments-</p><p>703 Molecular Devices, Sunnyvale, CA.) and data recorded at 200-300 kHz with a National </p><p>31 </p><p>704 Instrument USB-6356 D/A acquisition board and JClamp software (SciSoft). Vesicle </p><p>705 fusion was determined by monitoring membrane capacitance as a correlate of surface </p><p>706 area change. Capacitance was measured with a dual sinusoidal, FFT-based method </p><p>707 (Santos-Sacchi 2004). In this RC analysis method, two voltage frequencies, f1 and f2 </p><p>708 (twice the f1 frequency) are summed and the real and imaginary components of the </p><p>709 current response are used to determine the magnitudes of the three model components. </p><p>710 The time resolution of the Cm measurement is the period of f1, which we varied from </p><p>711 0.16 to 0.32 ms, corresponding to 6250 to 3125 Hz. Stimulation protocols were </p><p>712 performed after 10 minutes in the whole-cell mode to allow Ca2+ current run up and full </p><p>713 exchange of the internal solution into the cell (Schnee, Castellano-Munoz et al. 2011). A </p><p>714 dual sinusoid of 40 mV amplitude was delivered superimposed to the desired voltage </p><p>715 step. The mean IHC Cm was 10 ± 1 pF N = 25 and uncompensated series resistance of </p><p>716 10 ± 3 M. All voltages were corrected for a 4 mV junction potential. </p><p>717 Postsynaptic Recordings </p><p>718 Whole-cell voltage-clamp recordings were made at terminal endings of SGNs from P17-</p><p>719 21 animals (Grant, Yi et al. 2010). Cochlea were acutely dissected from the temporal </p><p>720 bone in chilled (~ 4°C) standard extracellular solution containing 1 mM curare. The </p><p>721 apical turn was excised after removing stria vascularis and tectorial membrane, and </p><p>722 placed under two dental floss fibers on a recording chamber filled with the standard </p><p>723 extracellular solution at room temperature. Recording pipettes were made from 1.5 mm, </p><p>724 thick-wall borosilicate glass (WPI). Pipettes were pulled using a multistep horizontal </p><p>725 puller (Sutter) and fire polished while applying a pressure to the back end of pipettes to </p><p>726 an impedance of 6-8 MΩ (Johnson, Forge et al. 2008). When whole-cell recording was </p><p>32 </p><p>727 achieved from a bouton, 5 µM TTX was perfused into the chamber, and EPSCs were </p><p>728 recorded in the standard solution (5.8 mM K+) for at least 3 min, then in 40 mM KCl for </p><p>729 1-5 min (depending on the duration of synaptic activity on each nerve). Standard </p><p>730 external solution contained (in mM): 5.8 KCl, 140 NaCl, 0.9 MgCl2, 1.3 CaCl2, 0.7 </p><p>731 NaH2PO4, 5.6 D-glucose, 10 HEPES, 2 Ascorbate, 2 Na-Pyruvate, 2 Creatine </p><p>732 Monohydrate, Osmolarity was 305 mosmls, pH = 7.4 (NaOH). Intracellular solutions </p><p>733 contained (in mM): 135 KCl, 3.5 MgCl2, 0.1 CaCl2, 5.0 EGTA, 5.0 HEPES, 2.5 Na2-ATP, </p><p>734 pH = 7.2 (KOH), osm ~290 mosmls. </p><p>735 Data Analysis: </p><p>736 Data were collected blinded to genotype for the experimentalist. Genotype was revealed </p><p>737 during analysis phase to ensure appropriate n values were obtained between groups. </p><p>738 Although gender was noted, no gender differences were observed between groups and </p><p>739 so data is presented combined. Data analysis was largely performed using unpaired </p><p>740 two-tailed t-tests unless otherwise stated. When non normal distributions were </p><p>741 observed, as in the EPSC histograms we selected different comparators as described </p><p>742 with the data. Similarly when large n values would bias comparisons, such as with </p><p>743 analyzing immunohistochemistry data, we moved to alternative more appropriate tests </p><p>744 as described in the text. Data is presented as mean ± standard deviation unless </p><p>745 otherwise stated. Sample size is presented in legends or in text. For physiology </p><p>746 experiments sample size reflects cell numbers which also indicates animal numbers as </p><p>747 we did not record from more than one cell in a preparation. For immunohistochemistry, </p><p>748 data is presented for animal numbers as well as image, cell and puncta numbers. </p><p>33 </p><p>749 The voltage against capacitance plots were arbitrarily fit with an exponential equation of </p><p>(-x(x-x )/t) 750 the form: Y = Y0 + A1 exp 0 where: Y0 is the baseline change in capacitance and is </p><p>751 near 0, A1 is the asymptote for the maximal change in capacitance, x0 is the voltage </p><p>752 where changes in capacitance measurements were first noted and t reflects the voltage-</p><p>753 dependence of the release (Cm/Vm). The equation for the Gaussian fit used in Fig. </p><p>(-2*((x-x )/w)^2) 754 6E is: Y = Y0 + (A/(w*√(π/2)))*exp c where Y0 is baseline, typically 0, A is the </p><p>755 area under the curve, w is the width and xc is the peak of the Gaussian. </p><p>756 Acknowledgments </p><p>757 This work was supported by National Institutes of Health, National Institute on Deafness </p><p>758 and Other Communication Disorders (NIDCD), Grants R01DC014712 to M.A.R.; M.A.R. </p><p>759 was also supported by an International Project Grant from Action on Hearing Loss. </p><p>760 NIDCD; F32 4F32 DC013721 grant from NIDCD to MN, Intramural Research Program </p><p>761 (Z01-DC000002) and NIDCD Advanced Imaging Core ZIC DC000081) to W.S. and </p><p>762 B.K.: RO1DC009913 to AJR, core grant P30 44992. We thank Autefeh Sajjadi for her </p><p>763 help with genotyping animals and basic husbandry. We thank Bill Roberts for comments </p><p>764 on the manuscript. </p><p>765 The authors declare no competing financial interests </p><p>766 References </p><p>767 Buran, B. N., N. Strenzke, A. Neef, E. D. Gundelfinger, T. Moser and M. C. Liberman </p><p>768 (2010). "Onset coding is degraded in auditory nerve fibers from mutant mice lacking </p><p>769 synaptic ribbons." J Neurosci 30(22): 7587-7597. </p><p>770 </p><p>34 </p><p>771 Chapochnikov, N. M., H. Takago, C. H. Huang, T. Pangrsic, D. Khimich, J. Neef, E. </p><p>772 Auge, F. Gottfert, S. W. Hell, C. Wichmann, F. Wolf and T. Moser (2014). "Uniquantal </p><p>773 release through a dynamic fusion pore is a candidate mechanism of hair cell </p><p>774 exocytosis." Neuron 83(6): 1389-1403. </p><p>775 </p><p>776 dick, o. (2001). "localization of the presynaptic cytomatrix protein piccolo at ribbon and </p><p>777 conventional synapses in the rat retina: comparison with basson." J Comp Neurol 439: </p><p>778 10. </p><p>779 </p><p>780 Farris, H. E., G. B. Wells and A. J. Ricci (2006). "Steady-state adaptation of </p><p>781 mechanotransduction modulates the resting potential of auditory hair cells, providing an </p><p>782 assay for endolymph [Ca2+]." J Neurosci 26(48): 12526-12536. </p><p>783 Fernandez, K. A., P. W. Jeffers, K. Lall, M. C. Liberman and S. G. Kujawa (2015). </p><p>784 "Aging after noise exposure: acceleration of cochlear synaptopathy in "recovered" ears." </p><p>785 J Neurosci 35(19): 7509-7520. </p><p>786 </p><p>787 Frank, T., M. A. Rutherford, N. Strenzke, A. Neef, T. Pangrsic, D. Khimich, A. Fejtova, </p><p>788 E. D. Gundelfinger, M. C. Liberman, B. Harke, K. E. Bryan, A. Lee, A. Egner, D. Riedel </p><p>789 and T. Moser (2010). "Bassoon and the synaptic ribbon organize Ca(2)+ channels and </p><p>790 vesicles to add release sites and promote refilling." Neuron 68(4): 724-738. </p><p>791 </p><p>792 Fuchs, P. A. (2005). "Time and intensity coding at the hair cell's <a href="/tags/Ribbon_synapse/" rel="tag">ribbon synapse</a>." J </p><p>793 Physiol 566(Pt 1): 7-12. </p><p>35 </p><p>794 </p><p>795 Furukawa, T. and S. Matsuura (1978). "Adaptive rundown of excitatory post-synaptic </p><p>796 potentials at synapses between hair cells and eight nerve fibres in the goldfish." J </p><p>797 Physiol 276: 193-209. </p><p>798 </p><p>799 Glowatzki, E. and P. A. Fuchs (2002). "Transmitter release at the hair cell ribbon </p><p>800 synapse." Nat Neurosci 5(2): 147-154. </p><p>801 </p><p>802 Grant, L., E. Yi and E. Glowatzki (2010). "Two modes of release shape the postsynaptic </p><p>803 response at the inner hair cell ribbon synapse." J Neurosci 30(12): 4210-4220. </p><p>804 Graydon, C. W., S. Cho, G. L. Li, B. Kachar and H. von Gersdorff (2011). "Sharp </p><p>805 Ca(2)(+) nanodomains beneath the ribbon promote highly synchronous multivesicular </p><p>806 release at hair cell synapses." J Neurosci 31(46): 16637-16650. </p><p>807 </p><p>808 Graydon, C. W., J. Zhang, N. W. Oesch, A. A. Sousa, R. D. Leapman and J. S. </p><p>809 Diamond (2014). "Passive diffusion as a mechanism underlying ribbon synapse vesicle </p><p>810 release and resupply." J Neurosci 34(27): 8948-8962. </p><p>811 </p><p>812 Jing, Z., M. A. Rutherford, H. Takago, T. Frank, A. Fejtova, D. Khimich, T. Moser and N. </p><p>813 Strenzke (2013). "Disruption of the presynaptic cytomatrix protein bassoon degrades </p><p>814 ribbon anchorage, multiquantal release, and sound encoding at the hair cell afferent </p><p>815 synapse." J Neurosci 33(10): 4456-4467. </p><p>816 </p><p>36 </p><p>817 Johnson, S. L., A. Forge, M. Knipper, S. Munkner and W. Marcotti (2008). "Tonotopic </p><p>818 variation in the calcium dependence of neurotransmitter release and vesicle pool </p><p>819 replenishment at mammalian auditory ribbon synapses." J Neurosci 28(30): 7670-7678. </p><p>820 </p><p>821 Johnson, S. L., H. J. Kennedy, M. C. Holley, R. Fettiplace and W. Marcotti (2012). "The </p><p>822 resting transducer current drives spontaneous activity in prehearing mammalian </p><p>823 cochlear inner hair cells." J Neurosci 32(31): 10479-10483. </p><p>824 </p><p>825 Kantardzhieva, A., M. C. Liberman and W. F. Sewell (2013). "Quantitative analysis of </p><p>826 ribbons, vesicles, and cisterns at the cat inner hair cell synapse: correlations with </p><p>827 spontaneous rate." J Comp Neurol 521(14): 3260-3271. </p><p>828 </p><p>829 Kantardzhieva, A., M. Peppi, W. S. Lane and W. F. Sewell (2012). "Protein composition </p><p>830 of immunoprecipitated synaptic ribbons." J Proteome Res 11(2): 1163-1174. </p><p>831 </p><p>832 Khimich, D., R. Nouvian, R. Pujol, S. tom Dieck, A. Egner, E. D. Gundelfinger and T. </p><p>833 Moser (2005). "Hair cell synaptic ribbons are essential for synchronous auditory </p><p>834 signalling." Nature 434(7035): 889-894. </p><p>835 </p><p>836 Kremer, J. R., D. N. Mastronarde and J. R. McIntosh (1996). "Computer visualization of </p><p>837 three-dimensional image data using IMOD." J Struct Biol 116(1): 71-76. </p><p>838 </p><p>37 </p><p>839 Li, G. L., S. Cho and H. von Gersdorff (2014). "Phase-locking precision is enhanced by </p><p>840 multiquantal release at an auditory hair cell ribbon synapse." Neuron 83(6): 1404-1417. </p><p>841 </p><p>842 Li, G. L., E. Keen, D. Andor-Ardo, A. J. Hudspeth and H. von Gersdorff (2009). "The </p><p>843 unitary event underlying multiquantal EPSCs at a hair cell's ribbon synapse." J Neurosci </p><p>844 29(23): 7558-7568. </p><p>845 </p><p>846 Lv, C., W. J. Stewart, O. Akanyeti, C. Frederick, J. Zhu, J. Santos-Sacchi, L. Sheets, J. </p><p>847 C. Liao and D. Zenisek (2016). "Synaptic Ribbons Require Ribeye for Electron Density, </p><p>848 Proper Synaptic Localization, and Recruitment of Calcium Channels." Cell Rep 15(12): </p><p>849 2784-2795. </p><p>850 </p><p>851 Mastronarde, D. N. (2005). "Automated electron microscope tomography using robust </p><p>852 prediction of specimen movements." J Struct Biol 152(1): 36-51. </p><p>853 </p><p>854 Matthews, G. and P. Fuchs (2010). "The diverse roles of ribbon synapses in sensory </p><p>855 neurotransmission." Nat Rev Neurosci 11(12): 812-822. </p><p>856 </p><p>857 Maxeiner, S., F. Luo, A. Tan, F. Schmitz and T. C. Sudhof (2016). "How to make a </p><p>858 synaptic ribbon: RIBEYE deletion abolishes ribbons in retinal synapses and disrupts </p><p>859 neurotransmitter release." Embo j 35(10): 1098-1114. </p><p>860 </p><p>38 </p><p>861 Nouvian, R., D. Beutner, T. D. Parsons and T. Moser (2006). "Structure and function of </p><p>862 the hair cell ribbon synapse." J Membr Biol 209(2-3): 153-165. </p><p>863 </p><p>864 Pangrsic, T., L. Lasarow, K. Reuter, H. Takago, M. Schwander, D. Riedel, T. Frank, L. </p><p>865 M. Tarantino, J. S. Bailey, N. Strenzke, N. Brose, U. Muller, E. Reisinger and T. Moser </p><p>866 (2010). "Hearing requires otoferlin-dependent efficient replenishment of synaptic </p><p>867 vesicles in hair cells." Nat Neurosci 13(7): 869-876. </p><p>868 </p><p>869 Parsons, T. D. (1994). "Calcium-triggered exocytosis and endocytosis in an isolated </p><p>870 presynaptic cell: capacitance measurements in sacucular hair cells." Neuron 13: 8. </p><p>871 Roberts, W. M. (1994). "Localization of calcium signals by a mobile calcium buffer in </p><p>872 frog saccular hair cells." J Neurosci 14(5 Pt 2): 3246-3262. </p><p>873 </p><p>874 Roux, I., S. Safieddine, R. Nouvian, M. Grati, M. C. Simmler, A. Bahloul, I. Perfettini, M. </p><p>875 Le Gall, P. Rostaing, G. Hamard, A. Triller, P. Avan, T. Moser and C. Petit (2006). </p><p>876 "Otoferlin, defective in a human deafness form, is essential for exocytosis at the </p><p>877 auditory ribbon synapse." Cell 127(2): 277-289. </p><p>878 </p><p>879 Rudolph, S., M. C. Tsai, H. von Gersdorff and J. I. Wadiche (2015). "The ubiquitous </p><p>880 nature of multivesicular release." Trends Neurosci 38(7): 428-438. </p><p>881 </p><p>39 </p><p>882 Rutherford, M. A., N. M. Chapochnikov and T. Moser (2012). "Spike encoding of </p><p>883 neurotransmitter release timing by spiral ganglion neurons of the cochlea." J Neurosci </p><p>884 32(14): 4773-4789. </p><p>885 </p><p>886 Santos-Sacchi, J. (2004). "Determination of cell capacitance using the exact empirical </p><p>887 solution of partial differential Y/partial differential Cm and its phase angle." Biophys J </p><p>888 87(1): 714-727. </p><p>889 </p><p>890 Schmitz, F., A. Konigstorfer and T. C. Sudhof (2000). "RIBEYE, a component of </p><p>891 synaptic ribbons: a protein's journey through evolution provides insight into synaptic </p><p>892 ribbon function." Neuron 28(3): 857-872. </p><p>893 </p><p>894 Schnee, M. E., M. Castellano-Munoz, J. H. Kong, J. Santos-Sacchi and A. J. Ricci </p><p>895 (2011). "Tracking vesicle fusion from hair cell ribbon synapses using a high frequency, </p><p>896 dual sine wave stimulus paradigm." Commun Integr Biol 4(6): 785-787. </p><p>897 </p><p>898 Schnee, M. E., M. Castellano-Munoz and A. J. Ricci (2013). "Response properties from </p><p>899 turtle auditory hair cell afferent fibers suggest spike generation is driven by </p><p>900 synchronized release both between and within synapses." J Neurophysiol 110(1): 204-</p><p>901 220. </p><p>902 </p><p>40 </p><p>903 Schnee, M. E., J. Santos-Sacchi, M. Castellano-Munoz, J. H. Kong and A. J. Ricci </p><p>904 (2011). "Calcium-dependent synaptic vesicle trafficking underlies indefatigable release </p><p>905 at the hair cell afferent fiber synapse." Neuron 70(2): 326-338. </p><p>906 </p><p>907 Snellman, J., B. Mehta, N. Babai, T. M. Bartoletti, W. Akmentin, A. Francis, G. </p><p>908 Matthews, W. Thoreson and D. Zenisek (2011). "Acute destruction of the synaptic </p><p>909 ribbon reveals a role for the ribbon in vesicle priming." Nat Neurosci 14(9): 1135-1141. </p><p>910 </p><p>911 Uthaiah, R. C. and A. J. Hudspeth (2010). "Molecular anatomy of the hair cell's ribbon </p><p>912 synapse." J Neurosci 30(37): 12387-12399. </p><p>913 </p><p>914 von Gersdorff, H., E. Vardi, G. Matthews and P. Sterling (1996). "Evidence that vesicles </p><p>915 on the synaptic ribbon of retinal bipolar neurons can be rapidly released." Neuron 16(6): </p><p>916 1221-1227. </p><p>917 </p><p>918 Zanazzi, G. and G. Matthews (2009). "The molecular architecture of ribbon presynaptic </p><p>919 terminals." Mol Neurobiol 39(2): 130-148. </p><p>920 </p><p>921 Zenisek, D., N. K. Horst, C. Merrifield, P. Sterling and G. Matthews (2004). "Visualizing </p><p>922 synaptic ribbons in the living cell." J Neurosci 24(44): 9752-9759. </p><p>923 </p><p>41 </p><p>924 Zheng, X. Y., D. Henderson, S. L. McFadden, D. L. Ding and R. J. Salvi (1999). </p><p>925 "Auditory nerve fiber responses following chronic cochlear de-efferentation." J Comp </p><p>926 Neurol 406(1): 72-86. </p><p>927 </p><p>928 Figure Legends </p><p>929 Figure 1. Number of synapses stays unaffected in ribbon less organs of corti. </p><p>930 A-D Max intensity projections of confocal z-sections of inner hair cells (IHCs) in apical </p><p>931 cochlea turns of P22 KO and wildtype littermates, immunolabeled for CtBP2/RIBEYE </p><p>932 (A), Homer (B), Bassoon (C) and Overlay (D). Virtually no punctated extra nuclear </p><p>933 CtBP2 staining can be seen in KO mice that is colocalized with other synaptic proteins, </p><p>934 while Bassoon and Homer appear generally unaffected. (E) 100 nm thick TEM sections </p><p>935 of pre- and postsynaptic densities in WT and KO mice at P28 confirm the absence of </p><p>936 electron dense ribbon structures in KO mice. (For E-G) Black arrows point to ribbon </p><p>937 structure, white arrows point to synaptic vesicles and white arrowheads point to the </p><p>938 remaining density resembling the arciform density. (F) Single slice from a 10 nm thick </p><p>939 tomographic section. (G) Reconstruction of complete synapses from data obtained used </p><p>940 to create E,F. Presynaptic density in cyan and postsynaptic density in green.Tethered </p><p>941 synaptic vesicles modeled in yellow, electron dense structure attributed to synaptic </p><p>942 ribbons in magenta. (H) Confocal max intensity projection of IHCs immunolabeled for </p><p>943 CtBP1 (green) and Homer (magenta). (I) Quantification of RIBEYE, Homer and </p><p>944 Bassoon puncta in the organ of corti normalized to the number of IHCs in analyzed </p><p>945 regions of interest, showing complete loss of synaptic RIBEYE staining in KO. The </p><p>42 </p><p>946 amount of Homer and Bassoon staining stays unaffected (p > 0.05). WT in blue, n = 20 </p><p>947 mice, quantified puncta: RIBEYE = 3796, Bassoon = 10215, Homer = 4340; KO in red, </p><p>948 n = 19 mice, n puncta: RIBEYE = 33, Bassoon = 10825, Homer = 4201. (J) Paired </p><p>949 synaptic puncta per IHC is lost in absence of RIBEYE staining (p ≤ 0.001) between WT </p><p>950 and KO. The number of synapses defined by colocated postsynaptic Homer and </p><p>951 presynaptic Bassoon puncta (p = 0.18) is unaffected in KO. (K) The number of extra </p><p>952 nuclear CtBP1 puncta is greatly reduced (p = 0.003) in KO mice. (WT in blue, n = 9 </p><p>953 mice, n puncta: CtBP1 = 19929, Homer 4940; KO in red, n = 7 mice, n puncta: CtBP1 = </p><p>954 11960, Homer = 5033). (L) Remaining CtBP1 puncta show significantly reduced </p><p>955 (p ≤ 0.001) pairing with postsynaptic Homer staining. Scale Bars: A-D, H: 5 µm; E, F: </p><p>956 100 nm. Boxes represent standard deviations of the mean. Significance levels of two </p><p>957 tailed unpaired t-tests: n.s., not significant; * p ≤ 0.05; ** p ≤ 0.01; *** p ≤ 0.001. </p><p>958 </p><p>959 Figure 1 Supplemental Figure 1: Immunohisotchemistry from WT (A) and KO (B) for </p><p>960 Bassoon (green) CTBP2 (red) and myosin VIIa (Blue). Low power images show </p><p>961 Bassoon is present in efferents of outer hair cells as well as presynaptically in IHCs. </p><p>962 Bassoon localization is much broader than RIBEYE’s and is minimally effected in the </p><p>963 mutant animal. </p><p>964 </p><p>965 Figure 2 Hearing in RIBEYE knockout mice. A Distortion products of otoacoustic </p><p>966 emissions (DPOAE) show no significant differences in the tested frequency range (4-</p><p>967 46 kHz in half octave increments) between KO (red, n = 30) and WT littermates (blue, </p><p>43 </p><p>968 n = 22), suggesting normal outer hair cell function. (B) Auditory brainstem response </p><p>969 (ABR) measurements in the tested frequency range (4-46 kHz in half octave </p><p>970 increments) reveal a small (≈ 10 dB SPL), but significant threshold shift in KO (red, n = </p><p>971 28) over their WT littermates (blue, n = 22) at P21-P22. (C) Mean ABR waveforms to </p><p>972 5 ms tone pips at 23 kHz at 60 dB SPL from KO (red, n = 30) and wild type littermates </p><p>973 (blue, n = 24) reveal a decrease in amplitude of the first ABR wave in KO. (D) </p><p>974 Quantification of peak to peak amplitude (wave I: p1-n1) differences between KO (red) </p><p>975 and WT littermates (blue) at 23 kHz were significant over most sound pressure levels </p><p>976 (20 dB SPL: p > 0.05; 30 & 60 dB SPL: p ≤ 0.01; 40-60 dB SPL: p ≤ 0.001) (E) </p><p>977 Amplitudes of first peak amplitudes normalized to their respective values at 60 dB SPL. </p><p>978 Amplitudes scale with their maximum value in WT and KO. (F) First peak latency (p1) is </p><p>979 not significantly altered in KO mice. For wave I amplitude and latency (D-F) analysis the </p><p>980 n-number varied between sound pressure levels by a clearly detectable first wave and </p><p>981 speaker calibration, n of WT/KO: 20 dB, 11/7; 30 dB, 21/11; 40 dB, 23/22; 50 dB, 23/26; </p><p>982 60 dB, 23/28; 70 dB, 5/6. Boxes represent standard deviations of the mean; whiskers </p><p>983 and shaded line in (C) represent standard errors of the mean. Significance levels of two </p><p>984 tailed unpaired t-tests: n.s., not significant; * p ≤ 0.05; ** p ≤ 0.01; *** p ≤ 0.001. </p><p>985 </p><p>986 Figure 3. Ribbonless synapses contain clusters of presynaptic voltage-gated Ca2+ </p><p>987 channels juxtaposed to postsynaptic glutamate receptors. A-D Max intensity </p><p>988 projections of airyscan z-sections of IHCs in apical cochlea turns of P35 KO and WT </p><p>989 littermates, immunolabeled for CtBP2/RIBEYE (A), GluA3 (B), CaV1.3 (C) and overlay </p><p>990 between calcium channels and glutamate receptors (D). Inner hair cell afferent </p><p>44 </p><p>991 synapses from WT have a synaptic ribbon: a cytoplasmic presynaptic density comprised </p><p>992 mainly of RIBEYE protein, recognized by anti-CtBP2 (green). Co-labelled are the </p><p>2+ 993 closely associated presynaptic voltage-gated Ca channels (CaV1.3, gray) and </p><p>994 postsynaptic AMPA receptors (GluA3, magenta). Inner hair cell afferent synapses from </p><p>995 KO lacked synaptic ribbons. The AMPA receptors in KO mice exhibited a ring-like </p><p>996 morphology, and the CaV1.3 channels clustered in the form of round spots or elongated </p><p>997 stripes, both similar to WT synapses. Scale bar: 2 µm </p><p>998 Figure 4. Changed size distribution but unaffected synapse number and </p><p>999 organization in ribbonless synapses (A) Quantification of RIBEYE, GluA3 and </p><p>1000 CaV1.3 puncta in the organ of corti normalized to the number of IHCs in analyzed </p><p>1001 regions of interest. The number of GluA3 receptor and CaV1.3 puncta is unaffected (p > </p><p>1002 0.05). WT in blue, n = 9 images from 3 mice, 95 cells quantified puncta: RIBEYE = </p><p>1003 1456, GluA3 = 1459, CaV1.3 = 1897 KO in red, n = 13 images from 3 mice, 248 cells, n </p><p>1004 puncta: RIBEYE = 28, GluA3 = 2149, CaV1.3 = 2681. (B) Colocalization of synaptic </p><p>1005 puncta per IHC. In absence of RIBEYE, the number of synapses, defined by </p><p>1006 colocalization of postsynaptic GluA3 and presynaptic CaV1.3, is unaffected (p = 0.13) in </p><p>1007 KO. (C) Volume of presynaptic CaV1.3, Bassoon and postsynaptic Homer and GluA3 in </p><p>1008 WT (blue, n synapses: CaV1.3 = 1370; Bassoon = 1917; Homer = 5219; GluA3 = </p><p>1009 1611) and KO (red, n synapses: CaV1.3 = 2132; Bassoon = 1817; Homer = 4990; </p><p>1010 GluA3 = 2334) mice. (D) Normalized cumulative distributions of volumes show </p><p>1011 increased sizes of postsynaptic GluA3 and Homer immunoreactivity. (C) Boxes </p><p>1012 represent standard deviations of the mean. Significance levels of two tailed unpaired t-</p><p>1013 tests (A/B) or two sample Kolmogorov-Smirnov test (C): D represents the maximum </p><p>45 </p><p>1014 distance of the Kolmogorov-Smirnov distribution functions. n.s., not significant; * p ≤ </p><p>1015 0.05; ** p ≤ 0.01; *** p ≤ 0.001; **** p ≤ 0.0001. </p><p>1016 Figure 5: No differences were observed in calcium or potassium currents </p><p>1017 between KO and WT animals. Ca2+ currents were obtained from mice ranging in age </p><p>1018 from P10-P12 for WT (A, blue) and P10-13 KO (B, red), with time course of stimulus </p><p>1019 shown above, are comparable in all measured properties. Voltage-current plots, </p><p>1020 presented in (C) as mean ± SD show no difference (n provided in figure). Summaries of </p><p>1021 peak current (D) and voltage of half activation (V1/2, E) are not statistically different (n= </p><p>1022 18 and 26 for WT and KO, p = 0.13 for peak current and 0.326 for V1/2). Potassium </p><p>1023 currents, obtained from responses to 50 ms voltage pulses ranging from -124 mV to 16 </p><p>1024 mV in 10 mV increments, from a holding potential of -84 mV are presented in (F) for WT </p><p>1025 and (G) for KO mice at P24. A summary of the half activation voltage as estimated from </p><p>1026 a Boltzmann fit to the tail current voltage-current (H) found no statistical difference (n = </p><p>1027 6 and 8 for WT and KO, respectively with p = 0.084). Current-clamp measurements </p><p>1028 found no difference in resting potential (I) (n = 5 and 8 for WT and KO, respectively, p = </p><p>1029 0.69). </p><p>1030 </p><p>1031 Figure 6. Capacitance responses recorded from RIBEYE knockout mice show </p><p>1032 little difference between phenotypes. Example records of Cm responses (top), </p><p>1033 showing two release components and Ca2+ currents (below) recorded from a WT (blue) </p><p>1034 (A) and KO (red) (B) in response to 1s depolarizations from -84 mV to -49 and -44 mV. </p><p>1035 Dashed line indicates pre-stimulus baseline Cm. Black solid line indicates the 1st linear </p><p>1036 component of release and cyan line the 2nd, superlinear component. (C) Summary plot </p><p>46 </p><p>1037 of 1st and 2nd component magnitudes. No statistical difference was found between </p><p>1038 groups. For the linear component n = 15 for WT and 15 for KO, p = 0.23. For 2nd, </p><p>1039 superlinear component n = 17 for both groups and p = 0.38. Filled stars indicate mean </p><p>1040 for this and all subsequent figures. (D) Summary plot of 1st and 2nd component rates </p><p>1041 obtained by linear fits to data points, as indicated by lines in (A,B). No statistical </p><p>1042 difference was found between groups where, n = 16 for WT amd 13 for KO, with p = </p><p>1043 0.266. For the second component rate, n = 17 for both, with p = 0.085. (D). (E) presents </p><p>1044 a population histogram for the voltage at which the superlinear response is first </p><p>1045 observed. Fits to Gaussian distributions show no change in the mean value but an </p><p>1046 increase in the width of the plot for the KO. (F) Example of delay in onset of capacitance </p><p>1047 change with small depolarizations for a KO cell. Plots of Cm verses voltage responses </p><p>1048 measured at 0.1 (G), 0.5 seconds (H) 1 sec (I) or 3 sec (J) show a significant difference </p><p>1049 for release at 0.1s and -44 mV (p=0.03), error bars represent SEM. Fitting the </p><p>1050 relationships with simple exponential functions reveal differences in the shapes of the </p><p>1051 curves but not in maximum release for the 0.1 and 0.5 plots but not the 1 and 3 sec time </p><p>1052 points. N provided in figure for each group. (K) Shows time to release is significantly </p><p>1053 longer for KO compared to WT for depolarizations eliciting less than 50% of the </p><p>1054 maximal calcium current; n = 32 for WT and 26 for KO, p = 0.0012. </p><p>1055 Figure 7. Changing internal Ca2+ buffering does not distinguish phenotypes. (A) </p><p>1056 Example records of Cm changes in response to 1s depolarizations from -49 mV in 0.1 </p><p>1057 EGTA and -39 mV in 1 and 5 mM EGTA for WT (blue) and KO (red). (B) Plot of the </p><p>1058 change in Cm in response to 1s voltage steps of -49, -44 and -29 mV for 0.1 EGTA and </p><p>1059 -49,-44,-39 and -34 mV for 5 EGTA, n provided in figure. (C) The time to the 2nd </p><p>47 </p><p>1060 component increases significantly with elevated Ca2+ buffer (p value indicated in figure) </p><p>1061 but is similar between phenotypes; for 0.1 EGTA n = 7 for WT and 6 KO , p = 0.93, </p><p>1062 steps to -29 mV for WT and KO, for 1 EGTA n = 15 for WT and13 for KO , with p = -</p><p>1063 0.13, steps to -36 + 8 mV WT and -37 + 9 mV KO and for 5 EGTA n = 3 for WT and 4 </p><p>1064 for KO, with p = 0.67, steps to -39 + 5 mV WT and -35 + 2 mV KO. (D) The rate of </p><p>1065 release to the -49 mV step declines equally between phenotypes with increasing </p><p>1066 [EGTA] for 0.1 EGTA, n = 7 for WT and 6 for KO, p = 0.323, for 1 EGTA n = 20 for WT </p><p>1067 and 22 for KO, p = 0.99 and for 5 EGTA n = 7 for WT and 6 for KO, p = 0.235. (E,F) </p><p>1068 Provide box plots of the first component of release for depolarizations to -49 mV (E) or -</p><p>1069 44 mV (F). Effects of buffers were similar between the two groups. </p><p>1070 Figure 8: Subtle differences exist between WT and KO mice postsynaptic </p><p>1071 properties. Postsynaptic bouton recordings were obtained from WT or KO in control </p><p>1072 and during application of 40 mM K+ to the bath. Hair cell recordings demonstrate similar </p><p>1073 depolarizations in WT and KO, suggesting that any differences in response properties </p><p>1074 may be synapticaly mediated. (A). Cells rapidly recovered following washout of K+. The </p><p>1075 solid line below the trace indicates the duration of the application. </p><p>1076 Postsynaptic recordings from afferent boutons in response to 40 mM K+ external </p><p>1077 solution application are presented in (B) for WT and (C) for KO with a sustained </p><p>1078 response and (D) for KO with a transient response. Recordings were made in the </p><p>1079 presence of 5 µM tetrodotoxin (TTX). Frequency responses were generated using a 1 </p><p>1080 second window sliding at 100 ms intervals (E,F). Populations were defined as transient </p><p>1081 or sustained by whether firing persisted after 20 seconds. Number of each response </p><p>1082 type is presented as fraction in each panel. Maximum frequency responses are </p><p>48 </p><p>1083 presented in box plot format with mean value shown as start (G); n = 10 for WT and 18 </p><p>1084 for KO, p = 0.313. The time to peak is presented in (H) where onset of K+ application </p><p>1085 response was defined as the time point when the frequency exceeded the spontaneous </p><p>1086 frequency +3x SD for two overlapping time windows (200 ms). The time to peak was </p><p>1087 statistically different when the one outlier was removed (time point at 181 sec); n = 8 for </p><p>1088 WT and 10 for KO. Time to peak only includes fibers whose peak frequency reached 30 </p><p>1089 Hz because those lower had too few points to make an accurate measure. (I) </p><p>1090 Integrating the frequency response over 50 seconds shows that WT release in a more </p><p>1091 sustainable manner than the KO, n = 8 for WT and 10 for KO, p = 0.021. Representative </p><p>1092 probability density functions for EPSC amplitudes are presented for WT number of </p><p>1093 events = 6583 WT and 1551 KO in (J) and KO in (K). Solid lines represent fits to either </p><p>1094 double Gaussian functions or Gaussian and gamma functions (smaller peak always fit </p><p>1095 with Gaussian function). The curve fitting the small events were integrated to measure </p><p>1096 the % of events under the small peak (shown next to each example). Only nerves that </p><p>1097 had at least 100 events are included to ensure robustness of the fitting. (L) presents a </p><p>1098 box plot of the WT and KO percent of area contributed by the small peak. Comparisons </p><p>1099 using a t-test for unequal variance (Welch test) suggest a significant, p = 0.032, n = 7 </p><p>1100 for WT and 13 for KO. (M) presents a box plot comparing the percentages of simple </p><p>1101 events, defined as single rise and decay times plots are not significantly different, n = 7 </p><p>1102 for WT and 13 for KO, p = 0.3. (N) provides box plots of the median amplitude, where </p><p>1103 no significant difference was observed, n = 7 for WT and 13 for KO for each, p = 0.105, </p><p>1104 while (O) are box plots of the coefficient of variation for the distributions in (N). Plots </p><p>1105 were different, p = 0.017, when equal variance was not assumed. </p><p>49 </p><p>1106 Figure 9: KO synapses have a larger population of small slow EPSCs. 7 WT </p><p>1107 including 15,564 events and 13 KO fibers including 17210 events were analyzed. (A) </p><p>1108 shows examples of EPSCs that range in amplitude and kinetics. The inset highlights </p><p>1109 one of the small slower EPSCs identified in KO fibers. Median decay times (B) and the </p><p>1110 Coefficient of Variation for the distribution in decay times (C) are presented as box plots. </p><p>1111 Neither population show statistical significance. (D) plots the skewness of the </p><p>1112 distributions of decay times and demonstrates that the KO decay time is significantly </p><p>1113 greater (away from 0), suggesting that the mean is larger than median, largely because </p><p>1114 they have a long tail of large decay time. (E), plots the EPSC decay time constant for </p><p>1115 every fiber (each fiber a different color) for both WT and KO. (F), summarizes the data </p><p>1116 from C as average decay time constant with EPSCs binned over 10 pA. (G), pooling </p><p>1117 fibers for WT and KO recordings allowed for the generation of a probability density </p><p>1118 function for event duration. These distributions are significantly different by KS test </p><p>1119 (p<10 -18). KS test (p<10-18). (H) is the cumulative probability function for the duration of </p><p>1120 EPSCs. Dashed lines are the individual fiber distributions. Solid red and Blue lines </p><p>1121 represent the population aggregate (summed responses) for KO and WT respectively. </p><p>1122 The cyan and magenta lines are the averages for WT and KO respectively. </p><p>1123 </p><p>1124 </p><p>1125 </p><p>1126 </p><p>1127 </p><p>50 </p><p>1128 </p><p>1129 </p><p>1130 </p><p>1131 </p><p>1132 </p><p>1133 </p><p>1134 </p><p>1135 </p><p>1136 </p><p>1137 </p><p>1138 </p><p>1139 </p><p>1140 </p><p>1141 </p><p>51 </p>
Details
-
File Typepdf
-
Upload Time-
-
Content LanguagesEnglish
-
Upload UserAnonymous/Not logged-in
-
File Pages0 Page
-
File Size-