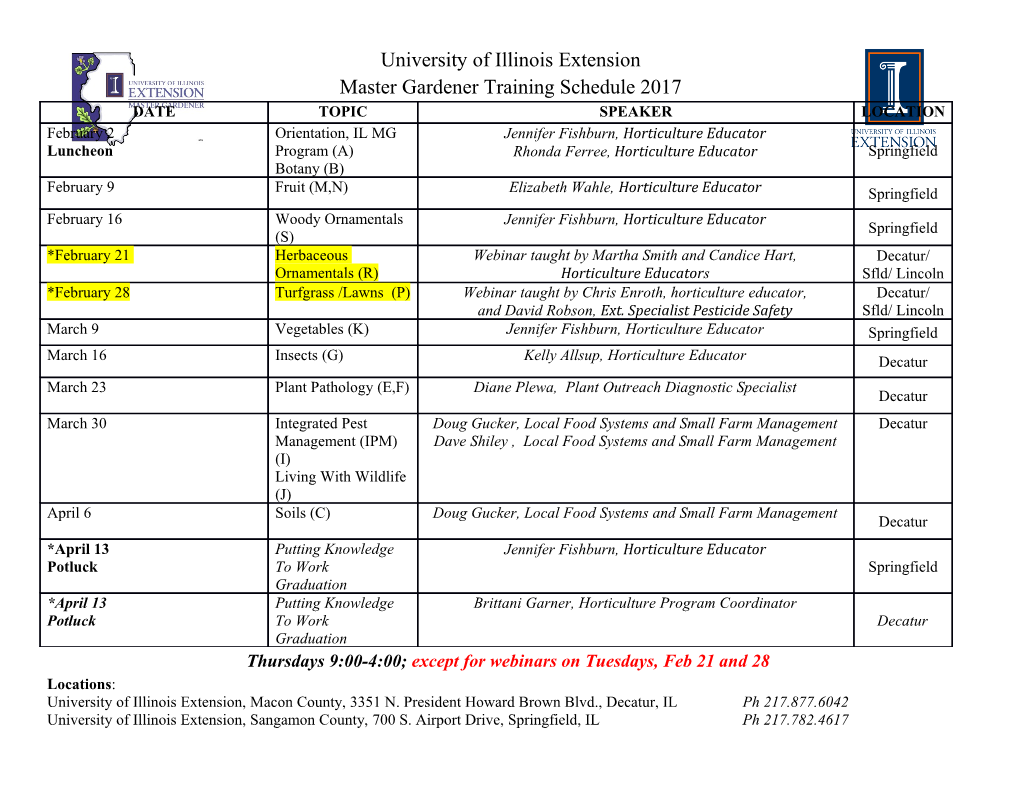
An arsenic-driven pump for invisible gold in hydrothermal systems G.S. Pokrovski, C. Escoda, M. Blanchard, D. Testemale, J.-L. Hazemann, S. Gouy, M.A. Kokh, M.-C. Boiron, F. de Parseval, T. Aigouy, L. Menjot, P. de Parseval, O. Proux, M. Rovezzi, D. Béziat, S. Salvi, K. Kouzmanov, T. Bartsch, R. Pöttgen, T. Doert Supplementary Information The Supplementary Information includes: 1. Materials: Description of Experimental and Natural Sulfarsenide Samples 2. Methods: Analytical, Spectroscopic and Theoretical Methods 3. Supplementary Text: Additional Notes on Thermodynamic Approaches Tables S-1 to S-11 Figures S-1 to S-12 Supplementary Information References Geochem. Persp. Let. (2021) 17, 39-44 | doi: 10.7185/geochemlet.2112 SI-1 1. Materials: Description of Experimental and Natural Sulfarsenide Samples 1.1. Experimental samples Three types of experiments at controlled laboratory conditions have been performed to produce synthetic Au-bearing sulfarsenide minerals (Tables S-1, S-2). Two hydrothermal types (MA, CE and LO series, Table S-1) in the presence of aqueous solution were conducted at temperature (T) of 450 °C and pressures (P) of 700–750 bar. The choice of these T-P parameters for hydrothermal MA, CE et LO series is dictated by the following reasons: i) they the pertinent to T-P conditions of hydrothermal ore deposits hosting sulfarsenide minerals in the Earth’s crust (~150–600 °C and <5 kbar) being roughly in the middle of the typical T-P window for magmatic porphyry Cu-Mo-Au and metamorphic Au ore deposits (e.g., Goldfarb et al., 2005; Kouzmanov and Pokrovski, 2012; Kolb et al., 2015; references therein); ii) the experimental T is sufficiently high to allow efficient chemical transfer and to reach fluid-mineral and mineral-mineral equilibrium within the intrinsically limited duration of laboratory experiments; and iii) the thermodynamic properties and related equations of state for aqueous and mineral species at these T-P conditions are sufficiently well constrained to date (see section 2.6 in this document) to enable thermodynamic validation of the Au speciation model proposed here. The third type (SF series, Table S-2) was conducted in a water-free system at 600 °C and pressures of <10 bar, following standard protocols of high-temperature ‘dry’ mineral synthesis widely used for sulfide minerals, to check whether the state of chemically bound gold would be the same as that in the presence of hydrothermal fluid. The first type of synthesis, hereafter called gold-capsule experiments, of most arsenopyrite (Apy) and löllingite (Lo) and some pyrite (Py) samples (MA and LO series, Table S-1) was based on the following formal reactions in aqueous solution: Fe2O3 + 4 As + 2 S = 2 FeAsS (Apy) + As2O3 (Eq. S-1) Fe2O3 + 6 As = 2 FeAs2 (Lo) + As2O3 (Eq. S-2) FeS + As = FeAsS (Apy) (Eq. S-3) Fe + 2 As = FeAs2 (Lo) (Eq. S-4) FeS + >0.9 S + <0.1 As ≈ FeS>1.9As<0.1 (Py) (Eq. S-5) Reactions (S-1) and (S-2), inspired from Wu and Delbove (1989) who succeeded to hydrothermally synthesise Au-rich arsenopyrite crystals hosting up to 17,000 ppm Au at 500 °C and 2 kbar, correspond to oxidizing conditions with high AsIII and low S–II activity in solution. In contrast, more direct reactions (S-3) and (S-4), correspond to more reducing conditions with lower AsIII and/or higher S–II activity, as demonstrated by thermodynamic modeling (Tables S-8 to S-10). The chosen reactions thus provide contrasting conditions in terms of oxygen fugacity, arsenic and sulfur activity and redox and the resulting gold solubility at equilibrium, but do yield the same thermodynamically stable arsenopyrite or löllingite phase. A couple of arsenian pyrite samples were also synthesised in this type of experiments based on reaction (S-5). Experiments were conducted in gold capsules (4 mm i.d., 4.4 mm o.d., 40 mm length), which were loaded with a ground mixture of reagent-grade powders of Fe2O3, FeS, As, Fe, and S (all >98 % purity, Sigma Aldrich) in the corresponding proportions matching the reaction stoichiometry and a 2 molal (mol/kg H2O) NaCl or NaOH aqueous solution, yielding a high solid/fluid mass ratio (~1:1), allowing efficient fluid-mineral equilibration, further favoured by the small sample chamber volume. The capsules were then welded shut and put in a titanium batch reactor, filled with water as a pressure medium (estimated by the degree of filling and PVT properties of H2O) and placed in a temperature controlled (±1 °C) oven. At the end of the run lasting respectively 30 and 73 to 81 days for the MA and LO series, the Geochem. Persp. Let. (2021) 17, 39-44 | doi: 10.7185/geochemlet.2112 SI-2 reactor was cooled down in water for 15 min, the capsules were opened and the solids were recovered, washed with hot water to remove precipitated salt and As2O3, dried at 50 °C and analysed as described in section 2. The quenched fluid phase could not be systematically recovered owing to its too small amounts (<0.1 mL) and partial loss during the capsule opening. The second type of hydrothermal synthesis, hereafter batch-reactor experiments (CE series), also conducted at 450 °C and ~700 bar, used titanium-alloy batch reactors of ~20 mL volume whose design and handling are described in detail elsewhere (Pokrovski et al., 2002a, 2019). This type of synthesis is based on reaction (S-3) for arsenopyrite, whereas for pyrite the starting solid was FeS2 that was directly reacted with a gold-bearing sulfidic solution with or without dissolved arsenic: FeS2 + <0.05 As(OH)3(aq) ≈ FeS>1.95As<0.05 (Eq. S-6) Compared to the gold-capsule experiments above, this type of synthesis enables accurate recovery and analyses of both solid and fluid phase, due to larger amounts of reagents and higher fluid/solid ratios (~10:1), but inevitably generates a lesser amount of data points. A weighed piece of gold foil (99.99 % purity, Heraeus) was attached to a titanium container suspended in the upper part of the reactor. A weighed amount of iron(II) disulfide (FeS2, 325 mesh, 99.8 % trace metals basis, Sigma Aldrich) for Py runs, or a stoichiometric 1:1 mixture of ground reagent-grade powders of FeS and As for Apy runs, was placed into the container, avoiding direct contact with the gold foil. The reactor was then loaded with a weighed amount of aqueous potassium thiosulfate solution with or without arsenious acid As(OH)3 (for Py runs) or with aqueous NaCl-HCl solution with addition of native sulfur to favour Au solubility (for Apy runs). These solution compositions were carefully chosen based on our previous experience (Pokrovski et al., 2019) and using thermodynamic modeling (see section 2.6) to ensure the stability of the dominant target phase in the experiment (Py or Apy) and, in the case of Py runs, large Au solubility due to the presence of both reduced and oxidised sulfur generated by thiosulfate breakdown (e.g., Pokrovski et al., 2015), to promote more efficient exchange of Au-bearing fluid with the preexisting FeS2. The reactors were placed in an oven; the pressure at the run temperature (±1 °C) was estimated from the degree of filling of the reactor (which is equivalent of the fluid density at experimental T-P) and the T-P-X-density properties of the well- known NaCl-H2O system (Driesner and Heinrich, 2007). These pressure estimations have an uncertainty of less than ±50 bar at 450 °C and total P value of 700 bar at our solution compositions (e.g., Pokrovski et al., 2009a). The run duration was up to 20 days for most experiments, which was considered long enough to attain the solid-fluid chemical equilibrium as shown by extensive previous experiments on Au solubility (Pokrovski et al., 2009a, 2015, 2019). At the end of the experiment, the reactor was placed in cold water, resulting in a fast separation of the container with the solid phase from the fluid that almost immediately condensed into liquid in the lower part of the reactor thus avoiding eventual fluid-solid reverse reactions during further cooling. The reactor was then cooled for 20 min, unloaded, and washed 3 times with hot aqua regia to remove all gold that was dissolved in the fluid at the run temperature but may partly have precipitated on reactor walls during cooling. The resulting washout solution was treated and analysed for Au. The solid phase was rinsed with water to remove traces of salt and dried at 50 °C. A third type of Apy and Py synthesis in a water-free system (SF series) was based on the reactions: Fe + As + S = FeAsS (Apy) (Eq. S-7) Fe + >1.9 S + <0.1 As ≈ FeS>1.9As<0.1 (Py) (Eq. S-8) This type of experiment that follows classical procedures of high-temperature mineral synthesis, has been performed at 600 °C in vacuumed flame-sealed fused silica tubes loaded with a piece of gold metal foil and ground mixtures of elemental Fe, As and S (purity >99 %, Sigma) in corresponding proportions (Table S-2) and a 1:1 NaCl-AlCl3 salt mixture as the fluxing reagent with a melting temperature of <500 °C. After 7 to 17 days from the experiment start, the tube was quenched in air, cut, and the solids were recovered and carefully washed with water to remove the salt and dried at 50 °C. Geochem. Persp. Let. (2021) 17, 39-44 | doi: 10.7185/geochemlet.2112 SI-3 The three methods of synthesis yielded well-crystalline fine-grained arsenopyrite, löllingite or pyrite as the major mineral phase with, in some cases, microscopic metallic gold particles and some minor impurities (<5 % of volume) of FeS, AsS, and salts (NaCl, As2O3), depending on the experiment, most of them likely having precipitated on cooling (Fig.
Details
-
File Typepdf
-
Upload Time-
-
Content LanguagesEnglish
-
Upload UserAnonymous/Not logged-in
-
File Pages43 Page
-
File Size-