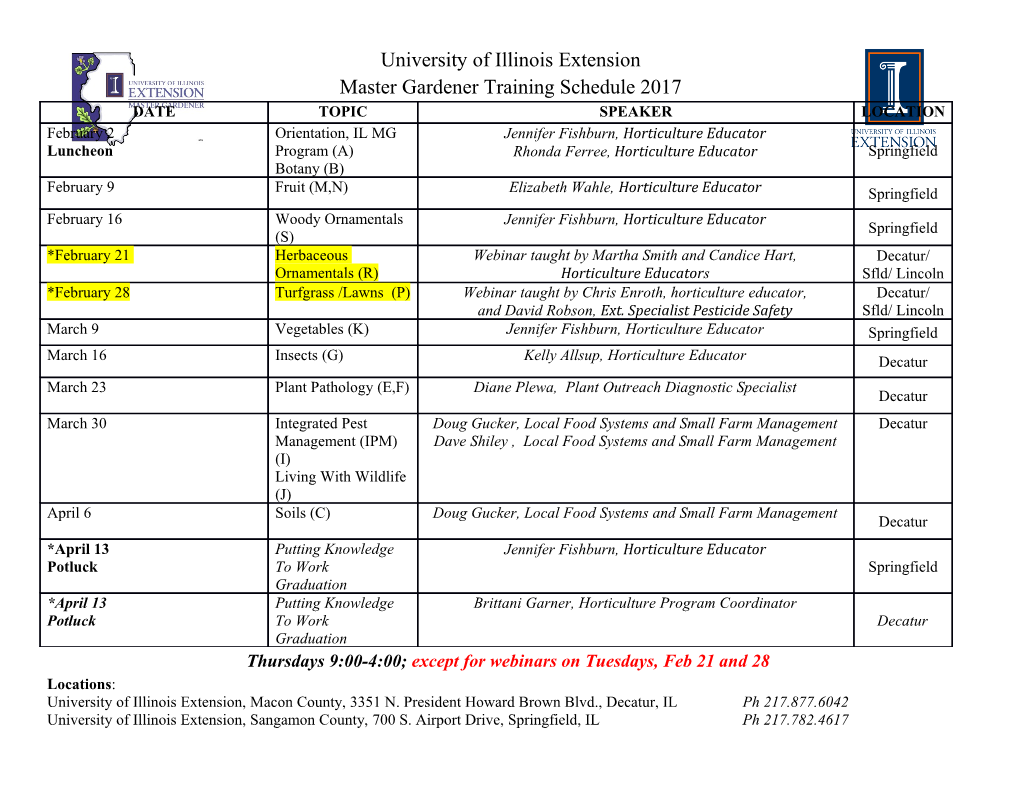
Department of Food Hygiene and Environmental Health Faculty of Veterinary Medicine University of Helsinki Helsinki, Finland STRESS RESPONSE MECHANISMS IN LISTERIA MONOCYTOGENES Mirjami Mattila ACADEMIC DISSERTATION To be presented, with the permission of the Faculty of Veterinary Medicine of the University of Helsinki, for public examination in Walter Auditorium of the EE-building (Agnes Sjöbergin katu 2, Helsinki), on the 10th of December 2020, at 12 noon. Helsinki 2020 Supervising Professor Professor Miia Lindström, DVM, Ph.D. Department of Food Hygiene and Environmental Health Faculty of Veterinary Medicine University of Helsinki Helsinki, Finland Supervisors Professor Emeritus Hannu Korkeala, DVM, Ph.D., M.Soc.Sc. Department of Food Hygiene and Environmental Health Faculty of Veterinary Medicine University of Helsinki Helsinki, Finland Professor Miia Lindström, DVM, Ph.D. Department of Food Hygiene and Environmental Health Faculty of Veterinary Medicine University of Helsinki Helsinki, Finland Reviewed by Director, Professor Aivars Bērziņš, DVM, Ph.D. National Institute of Food Safety, Animal Health and Environment “BIOR” Faculty of Veterinary Medicine Latvia University of Life Sciences and Technologies Riga, Latvia Professor Mati Roasto, DVM, M.Sc., Ph.D. Department of Food Hygiene and Veterinary Public Health Institute of Veterinary Medicine and Animal Sciences Estonian University of Life Sciences Tarto, Estonia Opponent Professor Emeritus Atte von Wright, M.Sc., Ph.D. Institute of Public Health and Clinical Nutrition University of Eastern Finland Kuopio, Finland ISBN 978-951-51-6847-4 (paperback) ISBN 978-951-51-6848-1 (PDF) Unigrafia Helsinki 2020 ABSTRACT Listeria monocytogenes is the causative agent of serious food-borne illness, listeriosis. The ability of L monocytogenes to survive and proliferate over a wide range of environmental conditions allows it to overcome various food preservation and safety barriers. To be able to control the risk of L. monocytogenes in the entire food chain, it is important to understand the mechanisms behind the stress tolerance of this pathogen. The aim of this study was to investigate the stress response mechanisms in L. monocytogenes strain EGD-e. Various cellular processes needed for growth and survival under unfavourable conditions are regulated through alternative sigma factors σB and σL. To gather deeper understanding about the role of these sigma factors at low temperatures, this study investigated the regulons of σB and σL, and the expression profile of the ΔsigBL double-mutant strain with a whole-genome expression analysis at 3°C and 37°C. Whole genome expression analysis revealed 198 and 86 genes positively regulated by σB during exponential growth at 3°C and 37°C, respectively. Altogether 29 genes were found to be under positive σB transcriptional regulation during exponential growth at both temperatures. At 3°C, 237 genes were detected to be under positive σL dependent transcriptional control, and at 37°C the number of down-regulated genes was 203. Only 47 of these genes exhibited positive σL transcriptional regulation at both temperatures. Of the 254 genes down-regulated in ΔsigBL at 3°C, 38% were detected also in ΔsigB or ΔsigL, and 62% were present only in ΔsigBL. At 37°C, 87% of the 139 down-regulated genes were present in either ΔsigB or ΔsigL and 13% were detected only in ΔsigBL. It thus appears that the growth at low temperature expands the specialized expression profile of ΔsigBL, but at optimal growth temperature the ΔsigBL expression profile resembles more the expression profiles of ΔsigB and ΔsigL. The phenotypic evaluation of the ∆sigB, ∆sigL, and ∆sigBL mutants revealed impaired growth in the presence of ethanol, and organic acids at low growth temperature. The growth of the sigB null mutant was compromised in the presence of lactic acid, acetic acid, and ethanol, while both the sigL and sigBL null mutants showed impaired growth in the presence of lactic acid, acetic acid, citric acid, and ethanol relative to the wild-type strain. The growth of the sigL and sigBL null mutants was also slower at low temperature (4°C) in defined medium relative to the parental strain. In addition, phenotypic microarray analysis exposed significant differences in growth of the wild-type strain and the sigB, sigL, and sigBL null mutant strains on different carbon sources and in the presence of various chemical compounds, such as antibiotics targeting cell wall, membrane, DNA, or protein synthesis. Apparently, the deletion in both sigB and sigL increases the sensitivity of the L. monocytogenes towards different stress conditions and chemical compounds and exposes phenotypic 3 characteristics absent from sigB or sigL null mutant strains. The stress response mechanisms positively regulated by both sigB and sigL might thus be more relevant than has been previously suspected. Overall, this study provided an expanded insight into σB and σL phenotypic roles and functional interactions in L. monocytogenes. DEAD-box proteins are conserved RNA helicases that can be found in most living organisms. They are needed in RNA metabolism and other metabolic processes and have been linked with cold stress tolerance in L. monocytogenes and many other bacteria. To investigate the role of DEAD-box RNA helicase encoding genes under low growth temperature, transcriptomic and phenotypic analyses of lmo0866, lmo1246, lmo1450, and lmo1722 deletion mutants were performed at 3°C, 25°C, and 37°C. The relative expression levels of lmo0866, lmo1246, lmo1450, and lmo1722 were significantly higher during growth at 3°C than at 37°C and also 30 min, 3 h, and 7 h after cold shock from 37°C to 5°C. At 3°C, the growth of ∆lmo0866 and ∆lmo1722 was totally impaired, and ∆lmo1450 showed only slight growth. The minimum growth temperatures of these mutants were significantly higher compared to the wild- type strain. The results suggest that lmo0866, lmo1450, and lmo1722 play an important role in the cold stress tolerance of L. monocytogenes. The temperature-dependent induction of flagella is a well-characterized phenomenon in L. monocytogenes. However, the essentiality of increased flagellum production during growth at low temperatures is still unclear. The role of flagella synthesis and motility genes flhA and motA in the cold stress tolerance of L. monocytogenes was studied at 3°C, 25°C, and 37°C. The relative expression levels of flhA and motA were found to be higher at 3°C compared to both 25°C and 37°C. The growth of the ΔflhA and ΔmotA deletion mutant strains was compromised at 3°C relative to the wild-type strain. These results illustrate that flhA and motA have a role in the cold stress tolerance of L. monocytogenes, yet the exact functions and regulation of these genes at low growth temperatures remains unknown. Deletion mutant ∆sigL was completely non-motile at 3°C and 10°C, and un-flagellated at 3°C. The ∆sigB and ∆sigBL mutants showed similar swarming pattern compared to the wild-type at 3°C, but the flagella formation of ∆sigBL was slightly compromised at 3°C compared to the wild-type. The cold-sensitive deletion mutant strains ∆lmo0866 and ∆lmo1450 were completely non-motile in BHI at 3°C, while the swarming pattern of the ∆lmo1246 was similar to the wild-type strain. The deletion mutant strains ΔflhA and ΔmotA were completely non-motile at 3°C and 25°C, and flagella formation was deficient. The growth of these strains was also significantly compromised when grown in BHI at 3°C. These findings suggest that the motility and flagella formation may play a role in the cold response of L. monocytogenes. 4 ACKNOWLEDGEMENTS This study was performed at the Department of Food Hygiene and Environmental Health, Faculty of Veterinary Medicine, University of Helsinki, and at the Finnish Centre of Excellence in Microbial Food Safety Research. The ABS Graduate School, Academy of Finland, Finnish Foundation for Veterinary Research, Walter Ehrström Foundation, Orion-Farmos Research Foundation, and the Medicine Fund of University of Helsinki are gratefully acknowledged for financial support. I express my deepest gratitude to my supervisors, Professor Emeritus Hannu Korkeala and Professor Miia Lindström for all their patience and encouragement during this project. Without their support and understanding it would not have been possible to complete this work after all these years. Professor Hannu Korkeala was the person who first introduced me to the fascinating world of food hygiene, as well as showed me how fun science can be! Professor Miia Lindström is warmly thanked for all the advice and expertise in both practical laboratory work and scientific writing. Docent Elina Säde is thanked for all the help during the final stage of the process. Professor Aivars Bērziņš and Professor Mati Roasto are are acknowledged for reviewing the thesis and Doctor Jennifer Rowland for revising the language. My warmest thanks to my co-authors in the original publications for their input and for sharing their vast knowledge with me. Panu Somervuo is specially thanked for the bioinformatics expertise throughout this work, and Taurai Tasara for pleasant and inspiring collaboration. Annukka Markkula, Roger Stephan, and Thomas Rattei are warmly thanked for their co-operation during this project. Riikka Keto-Timonen and Panu Somervuo are also thanked for acting as my support team. My dear colleague Henna Söderholm is warmly thanked, not only for the friendship that has lasted already more than half of my life, but also for her endless support,
Details
-
File Typepdf
-
Upload Time-
-
Content LanguagesEnglish
-
Upload UserAnonymous/Not logged-in
-
File Pages88 Page
-
File Size-