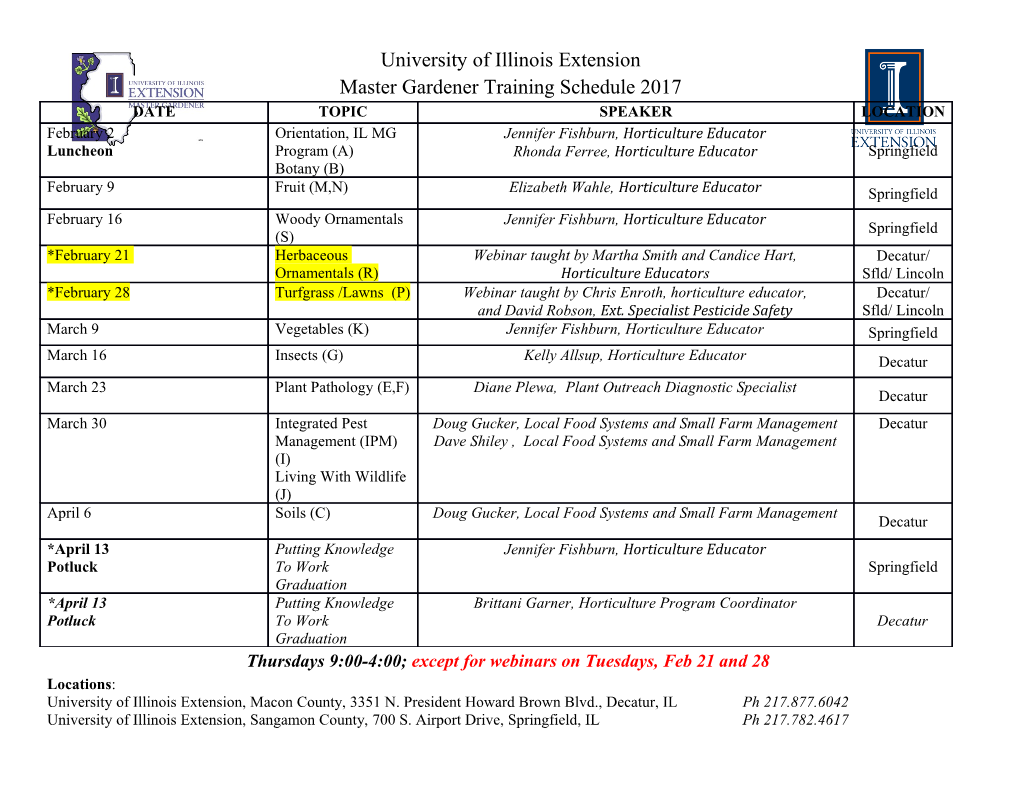
Action{angle variables in galactic dynamics Eugene Vasiliev Institute of Astronomy, Cambridge Summer School on Galactic Dynamics, Shanghai, June 2019 Hamiltonian mechanics Consider a particle moving in a potential Φ(x). x(t); v(t) are \ordinary" D-dimensional position/velocity coordinates; 1 2 H(x; v) = Φ(x) + 2 jvj is the Hamiltonian. The equations of motion are dx dv @Φ ≡ x_ = v; ≡ v_ = − dt dt @x One may consider a general class of Hamiltonian systems defined by H(q; p) as a function of generalized phase-space coordinates, which satisfy the Hamilton's equations of motion: @H @H q_ = ; p_ = − @p @q Poisson brackets Define the commutator operator for two functions of phase-space coordinates A(q; p) and B(q; p) as @A @B @A @B [A; B] ≡ − : @q @p @p @q It follows immediately that [A; A] = 0; [A; B] = −[B; A]; (antisymmetry) [A; B]; C + [B; C]; A + [C; A]; B = 0; (Jacobi identity) [qi ; qj ] = 0; [pi ; pj ] = 0; [qi ; pj ] = δij ; i; j = 1::D; and the Hamilton equations can be written as q_ i = [qi ; H]; p_ i = [pi ; H] Integrals of motion If [A; B] = 0, we say that A commutes with B. If a function A(q; p) commutes with the Hamiltonian, it is conserved along the particle's trajectory { we call it an integral of motion: dA @A dq @A dp = + dt @q dt @p dt @A @H @A @H = − @q @p @p @q = [A; H] = 0 Obviously, the Hamiltonian itself is an integral of motion. Phase-space distribution function f (q; p) satisfies the collisionless Boltzmann equation and hence is also conserved along the trajectory of any particle. Canonical transformations Consider a change of variables from p; q to P; Q, and express the Hamiltonian H(P; Q) or any other function in phase space in terms of the new variables. If the new variables satisfy the canonical commutatation relations [Qi ; Qj ] = 0; [Pi ; Pj ] = 0; [Qi ; Pj ] = δij , such transformation is called canonical (or symplectic). It also preserves I Hamilton's equations of motion: _ _ Qi = [Qi ; H]; Pi = [Pi ; H]; I more generally, all Poisson brackets: A(p; q); B(p; q) = A(P; Q); B(P; Q); H I all Poincar´einvariants: p · dq I 2D-dimensional phase volume element: d D q d D p = d D Q d D P : F (q; Q) = q · Q F (q; P) = Q(q) · P One powerful way of constructing such transformations is to introduce @F @F a generating function F (q; P) such that p = ; Q = ; @q @P F could also be expressed in terms of some other combination of old and new variables, e.g., F (q; Q), etc. Examples of canonical transformations 1. Exchange: Q = p; P = q (i.e., there is no fundamental difference between coordinate and momentum variables). 2. Point transformation: define Q(q) in whatever way, and then P(q; p) is uniquely specified. For instance, cartesian to polar coordinates: q ≡ fx; yg to Q ≡ fr; φg implies P ≡ fpr ; pφg = (xpx + ypy )=r; xpy − ypx . 3. Hamiltonian flow: integrate the equations of motion for some time τ, and let fQ; Pg(q; p; τ) be the new coordinates and momenta of a point started from initial conditions q; p. Examples of canonical transformations 1. Exchange: Q = p; P = q : F (q; Q) = q · Q (i.e., there is no fundamental difference between coordinate and momentum variables). 2. Point transformation: define Q(q) in whatever way, and then P(q; p) is uniquely specified. F (q; P) = Q(q) · P For instance, cartesian to polar coordinates: q ≡ fx; yg to Q ≡ fr; φg implies P ≡ fpr ; pφg = (xpx + ypy )=r; xpy − ypx . 3. Hamiltonian flow: integrate the equations of motion for some time τ, and let fQ; Pg(q; p; τ) be the new coordinates and momenta of a point started from initial conditions q; p. One powerful way of constructing such transformations is to introduce @F @F a generating function F (q; P) such that p = ; Q = ; @q @P F could also be expressed in terms of some other combination of old and new variables, e.g., F (q; Q), etc. A free particle! 1 2 H(q; p) = 2 jpj =) pi (t) = const; qi (t) = pi t + const Unfortunately, it corresponds to an unbound motion, unlike [most] stars in galaxies. The next simplest (and more realistic) thing? Periodic motion: @H H(q; p) = H(p) =) pi (t) = const; qi (t) = t + const, @pi where q are treated as angle-like (periodic) variables, q + 2π ∼= q q2 on a D-dimensional torus. These are action{angle variables q1 The holy grail of Hamiltonian mechanics What is the simplest possible Hamiltonian system? The next simplest (and more realistic) thing? Periodic motion: @H H(q; p) = H(p) =) pi (t) = const; qi (t) = t + const, @pi where q are treated as angle-like (periodic) variables, q + 2π ∼= q q2 on a D-dimensional torus. These are action{angle variables q1 The holy grail of Hamiltonian mechanics What is the simplest possible Hamiltonian system? A free particle! 1 2 H(q; p) = 2 jpj =) pi (t) = const; qi (t) = pi t + const Unfortunately, it corresponds to an unbound motion, unlike [most] stars in galaxies. These are action{angle variables The holy grail of Hamiltonian mechanics What is the simplest possible Hamiltonian system? A free particle! 1 2 H(q; p) = 2 jpj =) pi (t) = const; qi (t) = pi t + const Unfortunately, it corresponds to an unbound motion, unlike [most] stars in galaxies. The next simplest (and more realistic) thing? Periodic motion: @H H(q; p) = H(p) =) pi (t) = const; qi (t) = t + const, @pi where q are treated as angle-like (periodic) variables, q + 2π ∼= q q2 on a D-dimensional torus. q1 The holy grail of Hamiltonian mechanics What is the simplest possible Hamiltonian system? A free particle! 1 2 H(q; p) = 2 jpj =) pi (t) = const; qi (t) = pi t + const Unfortunately, it corresponds to an unbound motion, unlike [most] stars in galaxies. The next simplest (and more realistic) thing? Periodic motion: @H H(q; p) = H(p) =) pi (t) = const; qi (t) = t + const, @pi where q are treated as angle-like (periodic) variables, q + 2π ∼= q q2 on a D-dimensional torus. These are action{angle variables q1 Integrability and the Arnold{Liouville theorem If I1 and I2 are two integrals of motion, then the Jacobi identity [I1; I2]; H + [I2; H]; I1 + [H; I1]; I2 = 0 implies that [I1; I2] is also an integral of motion. (Example: I1 = Lx ; I2 = Ly ) [I1; I2] = Lz ). If [I1; I2] is identically zero, the two integrals are said to be in involution. A Hamiltonian system with D degrees of freedom is integrable if it has D independent integrals of motion I1 ::: ID (including the Hamiltonian itself) which are all in involution with each other. The motion of any particle is restricted to a D-dimensional hypersurface of the 2D-dimensional phase space. Theorem: this hypersurface is diffeomorphic to (i.e., could be smoothly deformed into) a D-torus, parametrized by D periodic variables θ 2 [0::2π). Action{angle variables for a 1d simple harmonic oscillator 1 2 1 2 2 The simplest possible Hamiltonian system: H(q; p) = 2 p + 2 ! q . The trajectory is q(t) = A sin(!t + φ0); p(t) = A ! cos(!t + φ0), 1 2 2 and the energy is E = 2 ! A . The motion is periodic with frequency ! (, period 2π=!), so we define the angle θ = !t + φ0. The action J is 1 ×area enclosed by the trajectory: 2π p 1 I !A J = p dq 2π θ 1 Z 2π dq = p(θ) dθ 2π dθ A 0 q 1 Z 2π = A2! cos2 θ dθ 2π 0 A2! E = = 2 ! Action{angle variables for a 2d simple harmonic oscillator The same thing but in two dimensions: q = fx; yg; p = fpx ; py g; Hamiltonian: 1 1 H(q; p) = p2 + !2 x 2 + p2 + !2 y 2 2 x x 2 y y 0.5 ≡ Hx (x; px ) + Hy (y; py ) 0.4 ) 0.3 x ( Motion is separable in x; y { Φ 0.2 0.1 two uncoupled simple harmonic oscillators, 0.0 two integrals of motion Ex ; Ey , 0.4 actions are Jx = Ex =!x ; Jy = Ey =!y . 0.2 y 0 -0.2 -0.4 -0.6 -1 -0.5 0 0.5 1 0.1 0.3 0.5 x Φ(y) Action{angle variables for a 2d planar axisymmetric potential A slightly more complicated system: two degrees of freedom, motion in an axisymmetric potential Φ(x; y) = Φ(R), where R ≡ px 2 + y 2. Canonical coordinates: q = fR; φg; p = fpR ; pφg 1 p2 1 Hamiltonian: H = Φ(R) + p2 + φ ≡ Φ (R) + p2 2 R R2 eff 2 R p dΦ equations of motion: R_ = p ; φ_ = φ ; p_ = − eff ; p_ = 0 R R2 R dR φ integrals of motion: E and pφ 0.5 =0.6 Motion in R is described by a 1d E pφ 2 2 .4 effective potential Φeff (R) ≡ Φ(R) + p =R =0 φ 1.0 pφ 2 f . f 0 The radial action is e = Φ p φ 1 Z R+ 1.5 0 JR = pR (R; E; pφ) dR = π R− p φ Z R+ q 1 2.0 = 2 (E − Φeff (R) dR 0.0 0.2 0.4 0.6 0.8 1.0 1.2 π R− R Action{angle variables for a 2d planar axisymmetric potential Motion in φ:_pφ = 0 ) pφ = const, hence the azimuthal action is ) 2π 2 0.5 1 Z R 2 ( Jφ = pφ dφ = pφ.
Details
-
File Typepdf
-
Upload Time-
-
Content LanguagesEnglish
-
Upload UserAnonymous/Not logged-in
-
File Pages53 Page
-
File Size-