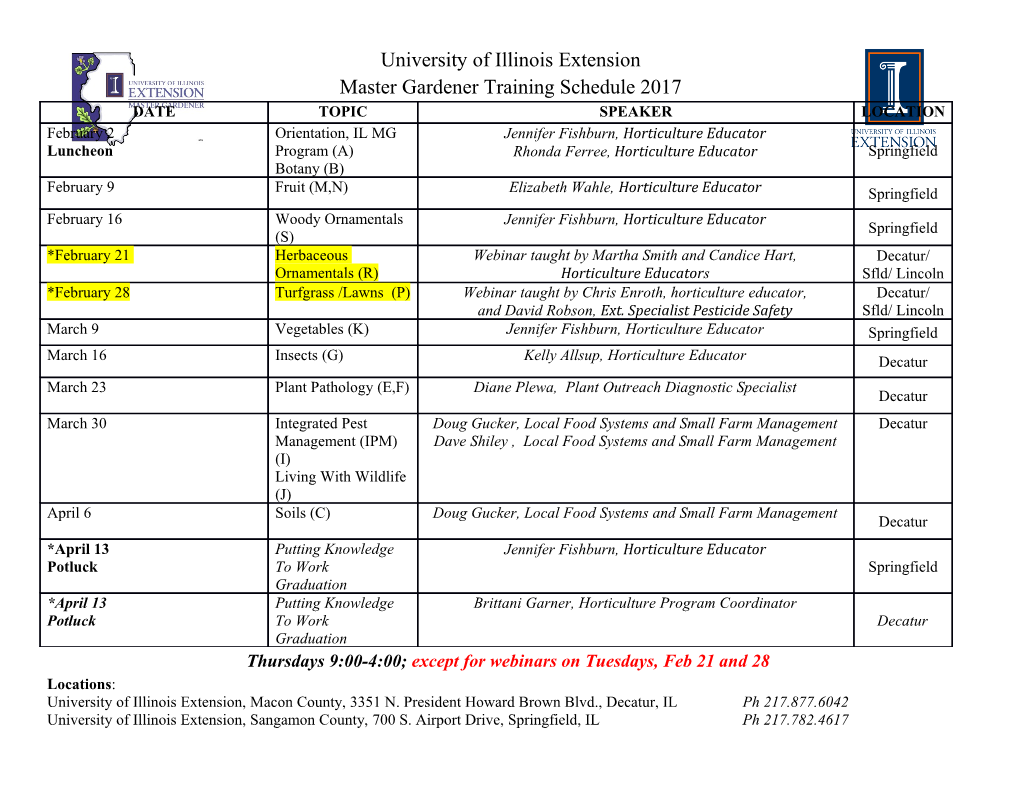
mathematics Article Tribonacci and Tribonacci-Lucas Sedenions Yüksel Soykan Department of Mathematics, Art and Science Faculty, Zonguldak Bülent Ecevit University, 67100 Zonguldak, Turkey; [email protected] Received: 14 November 2018; Accepted: 9 January 2019; Published: 11 January 2019 Abstract: The sedenions form a 16-dimensional Cayley-Dickson algebra. In this paper, we introduce the Tribonacci and Tribonacci-Lucas sedenions. Furthermore, we present some properties of these sedenions and derive relationships between them. Keywords: Tribonacci numbers; sedenions; Tribonacci sedenions; Tribonacci-Lucas sedenions MSC: 11B39; 11B83; 17A45; 05A15 1. Introduction The Tribonacci sequence fTngn≥0 and the Tribonacci-Lucas sequence fKngn≥0 are defined by the third-order recurrence relations: Tn = Tn−1 + Tn−2 + Tn−3, T0 = 0, T1 = 1, T2 = 1, (1) and: Kn = Kn−1 + Kn−2 + Kn−3, K0 = 3, K1 = 1, K2 = 3 (2) respectively. The Tribonacci concept was introduced by 14-year-old student M.Feinberg [1] in 1963. The basic properties of it were given in [2–12], and Binet’s formula for the nth number was given in [13]. The sequences fTngn≥0 and fKngn≥0 can be extended to negative subscripts by defining: T−n = −T−(n−1) − T−(n−2) + T−(n−3) and: K−n = −K−(n−1) − K−(n−2) + K−(n−3) for n = 1, 2, 3, . ., respectively. Therefore, recurrences (1) and (2) hold for all integers n. By writing Tn−1 = Tn−2 + Tn−3 + Tn−4, substituting for Tn−2 in (1), and eliminating Tn−2 and Tn−3 between this recurrence relation and the recurrence relation (1), a useful alternative recurrence relation is obtained for n ≥ 4 : Tn = 2Tn−1 − Tn−4, T0 = 0, T1 = T2 = 1, T3 = 2. (3) Extension of the definition of Tn to negative subscripts can be proven by writing the recurrence relation (3) as: T−n = 2T−n+3 − T−n+4. 2 Note that T−n = Tn−1 − Tn−2Tn (see [4]). Mathematics 2019, 7, 74; doi:10.3390/math7010074 www.mdpi.com/journal/mathematics Mathematics 2019, 7, 74 2 of 19 Next, we present the first few values of the Tribonacci and Tribonacci-Lucas numbers with positive and negative subscripts: n 0 1 2 3 4 5 6 7 8 9 10 . Tn 0 1 1 2 4 7 13 24 44 81 149 . T−n 0 0 1 −1 0 2 −3 1 4 −8 5 . Kn 3 1 3 7 11 21 39 71 131 241 443 . K−n 3 −1 −1 5 −5 −1 11 −15 3 23 −41 . It is well known that for all integers n, the usual Tribonacci and Tribonacci-Lucas numbers can be expressed using Binet’s formulas: an+1 bn+1 gn+1 T = + + (4) n (a − b)(a − g) (b − a)(b − g) (g − a)(g − b) and: n n n Kn = a + b + g respectively, where a, b, and g are the roots of the cubic equation x3 − x2 − x − 1 = 0. Moreover, p p p p 1 + 3 19 + 3 33 + 3 19 − 3 33 a = , 3 p p p p 1 + w 3 19 + 3 33 + w2 3 19 − 3 33 b = , 3 p p p p 1 + w2 3 19 + 3 33 + w 3 19 − 3 33 g = 3 where: p −1 + i 3 w = = exp(2pi/3), 2 is a primitive cube root of unity. Note that we have the following identities: a + b + g = 1, ab + ag + bg = −1, abg = 1. The generating functions for the Tribonacci sequence fTngn≥0 and Tribonacci-Lucas sequence fKngn≥0 are: ¥ x ¥ 3 − 2x − x2 T xn = and K xn = . ∑ n − − 2 − 3 ∑ n − − 2 − 3 n=0 1 x x x n=0 1 x x x We now present some properties of the Tribonacci and Tribonacci-Lucas numbers. • We have [3]: 0 1 Tn+1 Tn Tn−1 n B C N = @ Tn + Tn−1 Tn−1 + Tn−2 Tn−2 + Tn−3 A Tn Tn−1 Tn−2 and: n tr(N ) = Kn = Tn + 2Tn−1 + 3Tn−2 = 3Tn+1 − 2Tn − Tn−1, 2 2 2 Cn = −Tn + 2Tn−1 + 3Tn−2 − 2TnTn−1 + 2TnTn−2 + 4Tn−1Tn−2 Mathematics 2019, 7, 74 3 of 19 where: 0 1 1 1 0 B C N = @ 1 0 1 A , 1 0 0 tr(.) is the trace operator and Cn is defined by: n n n n n n Cn = a b + a g + b g which is the sum of the determinants of the principal minors of order two of Nn. • We have [4]: 3 2 2 Tn−1 − 1 = 2Tn−2Tn−1Tn + Tn−3Tn−1Tn+1 − Tn−2Tn+1 − Tn−3Tn 2 = Tn−2(2Tn−1Tn − Tn+1) + Tn−3(Tn − Tn−1Tn+1). • Tribonacci numbers satisfy the following equality [12]: Tk+n = TkKn − Tk−nCn + Tk−2n. In this paper, we define Tribonacci and Tribonacci-Lucas sedenions in the next section and give some properties of them. Before giving their definition, we present some information on Cayley-Dickson algebras. The algebras C (complex numbers), H (quaternions), and O (octonions) are real division algebras obtained from the real numbers R by a doubling procedure called the Cayley-Dickson process (construction). By doubling R (dim 20 = 1), we obtain the complex numbers C (dim 21 = 2); then, C yields the quaternions H (dim 22 = 4); and H produces octonions O (dim 23 = 8). The next doubling process applied to O then produces an algebra S (dim 24 = 16) called the sedenions. This doubling process can be extended beyond the sedenions to form what are known as the 2n-ions (see for example [14–16]). Next, we explain this doubling process. The Cayley-Dickson algebras are a sequence A0, A1, ... of non-associative R-algebras with involution. The term “conjugation” can be used to refer to the involution because it generalizes the usual conjugation on the complex numbers. For a full explanation of the basic properties of Cayley-Dickson algebras, see [14]. Cayley-Dickson algebras are defined inductively. We begin by defining A0 to be R. Given An−1, the algebra An is defined additively to be An−1 × An−1. Conjugation in An is defined by: (a, b) = (a, −b) multiplication is defined by: (a, b)(c, d) = (ac − db, da + bc) and addition is defined by componentwise as: (a, b) + (c, d) = (a + c, b + d). n p Note that An has dimension 2 as an R-vector space. If we set, as usual, kxk = Re(xx) for 2 x 2 An, then xx = xx = kxk . Now, suppose that B16 = fei 2 S : i = 0, 1, 2, ... , 15g is the basis for S, where e0 is the identity (or unit) and e1, e2,..., e15 are called imaginaries. Then, a sedenion S 2 S can be written as: 15 15 S = ∑ aiei = a0 + ∑ aiei i=0 i=1 Mathematics 2019, 7, 74 4 of 19 15 where a0, a1, ... , a15 are all real numbers. Here, a0 is called the real part of S, and ∑i=1 aiei is called its imaginary part. The addition of sedenions is defined as componentwise, and multiplication is defined as follows: if S1, S2 2 S, then we have: 15 ! 15 ! 15 S1S2 = ∑ aiei ∑ biei = ∑ aibj(eiej). (5) i=0 i=0 i,j=0 By setting i ≡ ei where i = 0, 1, 2, ... , 15, the multiplication rule of the base elements ei 2 B16 can be summarized as in the following Figure1 (see [17,18]). 0 1 2 3 4 5 6 7 8 9 10 11 12 13 14 15 0 0 1 2 3 4 5 6 7 8 9 10 11 12 13 14 15 1 1 − 0 3 − 2 5 − 4 − 7 6 9 − 8 − 11 10 − 13 12 15 − 14 2 2 − 3 − 0 1 6 7 − 4 − 5 10 11 − 8 − 9 − 14 − 15 12 13 3 3 2 − 1 − 0 7 − 6 5 − 4 11 − 10 9 − 8 − 15 14 − 13 12 4 4 − 5 − 6 − 7 − 0 1 2 3 12 13 14 − 14 − 8 − 9 − 10 − 11 5 5 4 − 7 6 − 1 − 0 − 3 2 13 − 12 15 15 9 − 8 11 − 10 6 6 7 4 − 5 − 2 3 − 0 − 1 14 − 15 − 12 − 12 10 − 11 − 8 9 7 7 − 6 5 4 − 3 − 2 1 − 0 15 14 − 13 13 11 10 − 9 − 8 8 8 − 9 − 10 − 11 − 12 − 13 − 14 − 15 − 0 1 2 2 4 5 6 7 9 9 8 − 11 10 − 13 12 15 − 14 − 1 − 0 − 3 3 − 5 4 7 − 6 10 10 11 8 − 9 − 14 − 15 12 13 − 2 3 − 0 − 1 − 6 − 7 4 5 11 11 − 10 9 8 − 15 14 − 13 12 − 3 − 2 1 − 0 − 7 6 − 5 4 12 12 13 14 15 8 − 9 10 − 11 − 4 5 6 7 − 0 − 1 − 2 − 3 13 13 − 12 15 − 14 9 8 11 − 10 − 5 − 4 7 − 6 1 − 0 3 − 2 14 14 − 15 − 12 13 10 − 11 8 9 − 6 − 7 − 4 5 2 − 3 − 0 1 15 15 14 − 13 − 12 11 10 9 8 − 7 6 − 5 − 4 3 2 − 1 − 0 Figure 1. Multiplication table for sedenions’ imaginary units. From the above table, we can see that: e0ei = eie0 = ei; eiei = −e0 for i 6= 0; eiej = −ejei for i 6= j and i, j 6= 0. The operations requiring the multiplication in (5) are quite a few. The computation of a sedenion multiplication (product) using the naive method requires 256 multiplications and 240 additions, while an algorithm, which was given in [19], can compute the same result in only 122 multiplications (or multipliers, in the hardware implementation case) and 298 additions (for more details, see [19]).
Details
-
File Typepdf
-
Upload Time-
-
Content LanguagesEnglish
-
Upload UserAnonymous/Not logged-in
-
File Pages19 Page
-
File Size-