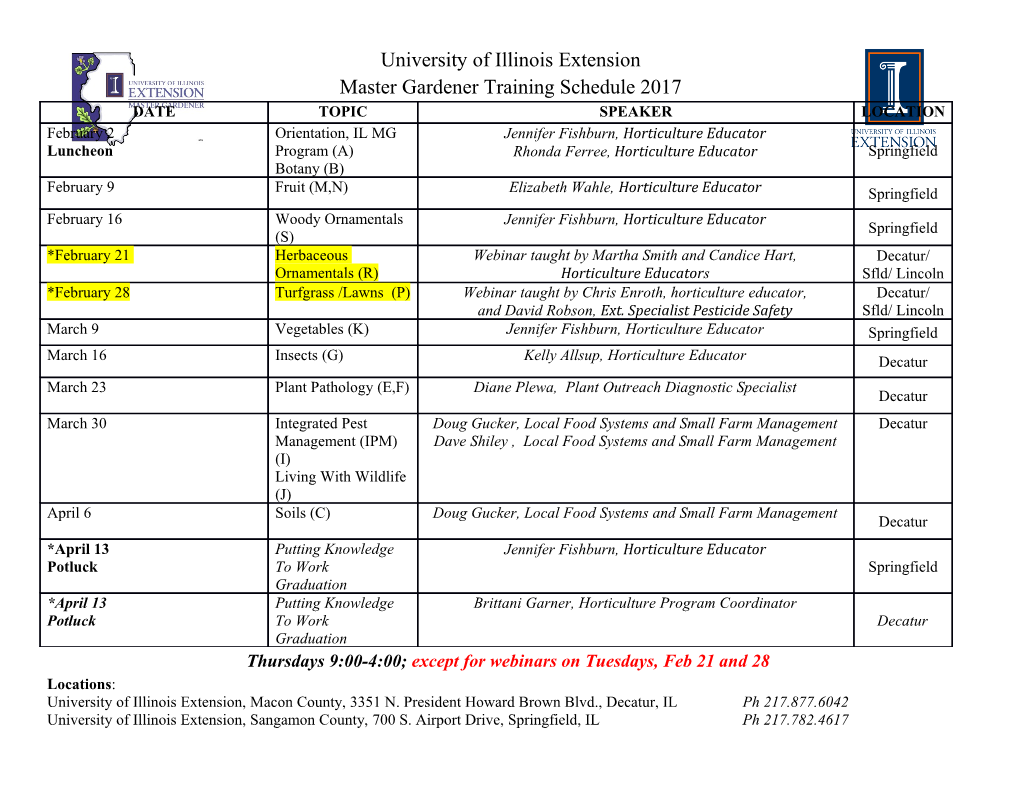
Development of Durable Ceramic Matrix Composite Turbine Components for Advanced Propulsion Engine Systems Dongming Zhu Durability and Protective Coatings Branch Structures and Materials Division NASA John H. Glenn Research Center Cleveland, Ohio 44135, USA 8th Pacific Rim Conference on Ceramic and Glass Technology Vancouver, May 31-June 5, 2009 1 Revolutionary Ceramic Coatings and Composites Greatly Impact Turbine Engine Technology — Ceramic thermal/environmental barrier coating (T/EBC) and ceramic matrix composite (CMC) development goals - Meet engine temperature and performance requirements - Ensure long-term durability - Develop design tools and lifing methodologies - Improve technology readiness — Crucial for envisioned supersonic vehicles: reduced engine emission, improved efficiency and long-term supersonic cruise durability 2 Revolutionary Ceramic Coatings and Composites Greatly Impact Turbine Engine Technology - Continued Step increase in the material’s temperature capability Temperature 3100°F SiC/SiC Capability (T/EBC) surface CMC combustor 2800ºF 3000°F+ (1650°C+) combustor 2700°F SiC/SiC CMC TBC 2700°F (1482C) turbine system Increase in T 2500ºF across T/EBC Turbine TBC Ceramic Matrix Composite 2400°F (1316°C) Single Crystal Superalloy 2000°F (1093°C) Gen. IV Gen III Gen II – Current commercial Gen I Year 3 MOTIVATION — Thermal and environmental barrier coatings with advanced hot-section substrate component materials help increase gas turbine operating temperatures, reduce cooling requirements, improve engine fuel efficiency and relia bility — Prime-reliant coating system is key to engine component durability Combustor Vane Blade Ceramic nozzles Temperature increase Turbine CMC combustor liner , vane/blade (a) Current TEBCs (b) Advanced T/EBCs 4 Advanced Ceramic Matrix Composite Turbine Airfoil Systems Emphasized in the Current Program — Advanced CMC turbine airfoil technology significantly improves engine performance by further increasing materials temperature capability, reducing engggine weight and coolin gqg requirements Gas TBC Bond Metal Gas TBC Bond Metal Gas EBC Bond CMC Gas EBC Bond CMC coat blade coat blade coat blade coat blade 3200 F 3200 F 2200 F (T41) (T41) 2900 F (T41) 2500 F TBCs 2700 F EBCs Tsurface 200 F Tsurface increase 300 F increase 2000-2200 F Tsurface EBCs Thin turbine Tsurface Baseline metal coating temperature development Thin turbine coating development Current metal turbine State of the art metal CMC HPT turbine CMC LPT turbine airfoil system turbine airfoil system airfoil system airfoil system 5 Outline ─ Research & development emphases and thrust areas ─ High h eat fl ux test ing met hod s f or CMC turbi ne ai rf oil development ─ Thermal and environmental barrier coatings systems development ─ Design tool and life prediction of coated CMC components ─ Summary and future directions 6 Research and Development Emphases and Thrust Areas (continued) Ӎ Advanced coating and CMC development and processing Ӎ Simulated engine heat-flux testing for coated component design and process validation High heat-flux thermal gradient mechanical testing and simulated engine testing of coated CMC specimens and subcomponents Advance d env ironmen ta l Embedded barrier coating development 2D Five-harness Satin TC Temperature 3D Orthogonal Angle Interlock SiC/BN nanotube () synthesis for Braid nano-composite coating Embedded thermocouple and applications CMC fiber architecture design heat flux sensor development and property modeling 7 Research and Development Emphases and Thrust Areas (continued) Ӎ Development of physics-based design tools and computational life models Materials and Advanced testing- systems simulating Database FEA So lver module Physical process Atomistic and Continuum and Mechanisms- modldels mo dldule micro-mechihanics structural environment models module mechanics interaction module module Design Tool and Life Prediction TC Test rig Simulated high pressure and high Design tool development for coated CMC velocity combustion flow and CMC turbine components turbine airfoil for heat transfer modeling 8 High-Heat-Flux Test Approaches – Turbine level high-heat-flux tests crucial for CMC coating system developments 2 • High power CO2 laser high-heat-flux rig (up to 315 W/cm ) Turbine: 450°F across 100 microns Combustor:1250°F across 400 microns Heat flux T om surface rr Distance f Test rig cooling 9 Thermal Conductivity Measurement by a Laser High-Heat- Flux Approach kceramic (t) qthru lceramic / Tceramic (t) Where l q dl l q dl q q q q T (t) T T bond thru substrate thru thru delivered reflected radiated and ceramic ceramic surafce metal back 0 0 kbond (T ) ksubstrate (T ) 8 m pyrometer qdelivered for Tceramic-surface qreflected qradiated ceramic coating bond coat Tceramic Tmeasured qthru Tsubstrate Tbond T tc subtbstra te Two-color and 8 Optional miniature m pyrometers for q thermocouple for thru additional heat-flux Tsubstrate-back calibration 10 Laser Heat Flux Testing in Water Vapor Environments for High Temperature SiC/SiC Ceramic Matrix Composites ─ High temperature and high-heat-flux testing capabilities ─ “Micro-steam environment” allowing high water vapor pressure, relatively high velocity under very high temperature condition - Steam injected at up to 5m/sec - Testing temperature >1700°C 11 High Pressure Burner Rig for Thermal and Environmental Barrier Coating Development ─ Realistic engine combustion environments for specimen and component testing High Pressure Burner rig (6 to 12 atm) Ve loc ity 900 m /s CtdCoated ceram itbiic turbine vane test fixtures 12 Thermal Conductivity of EBC Material Systems – Thermal conductivity of plasma-sprayed HfO2-(Y,Gd/Nd,Yb)2O3 systems determined using laser heat flux approaches 1.5 1.5 2.5 K K 2.0 1.0 1.0 1.5 ~k0 1.0 onductivity, W/m- onductivity, onductivity, W/m-K conductivity, W/m- cc cc 0.5 Tsurface=~3000°F 0.5 k20 Tinterface=~2012°F <k20> 0.5 <k0> Thermal k0 Thermal Thermal Thermal k(T) predicted k20 predicted 0.0 0.0 0.0 1000 1100 1200 1300 1400 1500 1600 1700 1800 1000 1100 1200 1300 1400 1500 0.0 5.0 10.0 15.0 20.0 Temperature, °C Average temperature, °C Time, hours 1.6 k0 K 1.4 k20 , W/m- yy 121.2 1.0 0.8 Low hermal conductivit Higher durability TT Possible optimum region conductivity region 0.6 region 10 12 14 16 18 20 22 Total dopant concentration, mol% 13 High-Heat-Flux Thermal Gradient Cyclic Testing of EBC Material Systems – Coating degradation modes can be monitored in real time K K 2.0 2.0 2000 Normalized kcera 1600 Normalized k 1.5 1.5 1500 tivity, W/m- tivity, W/m- C C cc 1200 cc 1.0 1.0 1000 800 Temperature, ° Temperature, ° thermal condu thermal Tsurface thermalcondu dd dd 050.5 Tinterface 400 050.5 Tsurface 500 Tback Tinterface Tback Normalize 0.0 0 Normalize 0.0 0 0 5 10 15 20 25 0.02.04.06.08.010.0 Time, hours Time, hours 14 Interface Reactions of Baseline Coating in Heat Flux and Water Vapor Cyclic Environments — Significant interfacial pore and eutectic phase formation due to the water vapor attack and Si diffusion under the thermal gradient cycling conditions at interface temperature 1300°C ZrO2-8wt%Y% 2O3 Mullite+BSAS Si SiC/SiC BSAS Mullite BSAS Mullite Si Si 15 Sintering and Creep Induced Failure of TEBCs ― Models used to predict long-term sintering behavior from sintering data ― Variable sintering rates observed - Initiallyyy very fast sinterin g - Reduced sintering rates with increasing time ― Sintering-creep can induce surface cracking and delamination Plasma-sprayed ZrO2-8wt%Y2O3 ZrO2-8wt%Y2O3/Mullite+BSAS/Si System 1200°C-e% 1200°C-de/dt 1300°C-e% 1300°C-de/dt 1400°C-e% 1400°C-de/dt 0.0 200 1500°C-e% 1500°C-de/dt 1.5 0.0 -0.2 2 1.0 150 G thru 0.5 -5.0 10-9 -0.4 1500 C G dela min n e=DL/L, % n e=DL/L, 1500 C , 1/sec % rains, e rate, J/m ii tt 000.0 tt -0.6 G thru 100 -1.0 10-8 1400C -0.5 E ~60GPa TBC -1.0 -0.8 Shrikage s Shrikage 50 -8 releas Energy -1.5 Strain rate-de/d -1.5 10 -1.0 ring shrinkage stra shrinkage ring Gdelamin G -2.0 1400C C Sinte -2.5 -2.0 10-8 -1.2 0 0 100 200 300 400 500 600 0 5 10 15 20 Time, hours Time, hours 16 Advanced Coating System Development — Mutli-component zirconia/hafnia-, perovskite and pyrochlore-oxide-based systems as high stability top coats for ceramic components: defect cluster coating concept demonstrated • Rare earth dopants for improved thermal stability • Transition metal dopants for phase stability and temperature capability of EBCs • Thin coating configurations emphasized for turbine application — Low stress, strain tolerant interlayer and high strength bond coat concepts • Advanced modified HfO2-aluminosilicates show high performance – Nano composite structures – Controlled thermal conductivity and thermal expansion – Controllable Si activity suitable for improved bonding and stability • Novel compositional and architectural design to achieve maximum energy dissipation and durability – Alternating composition layered coating (ACLC) concept demonstrated – Temperature Actuation Coating (TAC) systems 17 Advanced Thermal and Environmental Barrier Coatings for Si-based Ceramic Components – Advanced TEBC System (US Patent Appl. No. 11/510 573) • High stability HfO2 layer with graded interlayer, environmental barrier and advanced bond coats • Alternating composition layered and nano-composite coating interlayer • BSAS, alloyed mullite and rare earth silicate EBCs • OidOxide-Si compos ite bon d coats Low expansion HfO2 Interlayer: Compositional layer graded system Doped mullite-HfO2, with and rare earth silicate EBCs Ceramic composite bond coat HfO2 and
Details
-
File Typepdf
-
Upload Time-
-
Content LanguagesEnglish
-
Upload UserAnonymous/Not logged-in
-
File Pages35 Page
-
File Size-