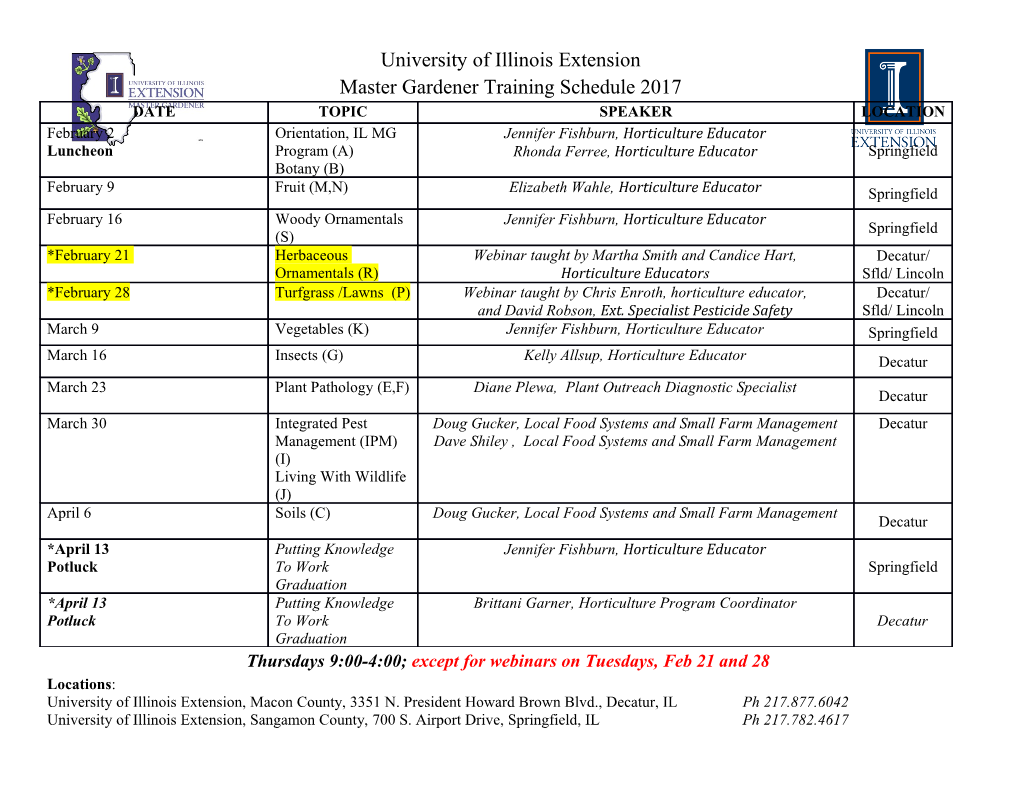
Master Thesis Investigation of Spent Nuclear Fuel Pool Coolability Fredrik Nimander Supervisor: Henryk Anglart Division of Nuclear Reactor Technology Royal Institute of Technology Stockholm, Sweden, August 2011 TRITA-FYS 2011:51 ISSN 0280-316X ISRN KTH/FYS/–11:51–SE ii ABSTRACT The natural catastrophe at Fukushima Dai-ichi 2011 enlightened the nuclear community. This master thesis reveals the non-negligible risks regarding the short term storage of spent nuclear fuel. The thesis has also investigated the possibility of using natural circulation of air in a passive safety system to cool the spent nuclear fuel pools. The results where conclusive: The temperature difference between the heated air and ambient air is far too low for natural circulation of air to remove any significant amount of heat from the spent nuclear fuel pool in a worst case scenario. Air, as with any gas, has too low density and a specific heat too low to be able to remove the heat generated by spent nuclear fuel shortly after it has been removed from the reactor core. The author does not deny the possibility of slightly prolonging the boiling with other designs. The author does however suggest other possibilities to prolong cooling with the conclusion that large enough spent fuel pools would constitute the simplest solution. iii iv Abstract ACKNOWLEDGEMENTS The author would like to thank Henryk Anglart for his help and supervision of the master thesis. He allowed the author to complete the master thesis during the summer of 2011 even though it is standard to write a thesis during a normal semester. The author also wants to thank PHD-student Roman Thiele for his help with OpenFoam. This software package is extremely useful and valuable, but it has a rather steep learning curve when getting started since the documentation is very general rather than detailed. Without the help of Roman there might not have been enough time to learn how to use this software package as a tool for the work that needed to be done. A special thanks goes to Nils-Gunnar Ohlson, teacher at KTH, for his help with finding the appropriate information on how to calculate the structural integrity of the copper wall designed for the passive safety system that was investigated for this thesis. v vi Acknowledgements NOMENCLATURE Symbol Dimensions Description Latin Symbols −1 −1 Cp Jkg K Specific heat E J Energy Esat J Energy required to saturate pool Evap J Energy reguired to evaporate water Gr - Grashof number h Wm−2K−1 Heat transfer coefficient k Wm−1K−1 Thermal conductivity Nu - Nusselt number P r - Prandtl number q00 Wm −2 Heat flux Q Js−1 Heat −1 Q0 Js Thermal Power of reactor during operation Ra - Rayleigh number s Pa Stress top s Continuous perational time of fuel in reactor ts s Time since reactor shutdown tsat s Time until spent fuel pool becomes saturated T K Temperature Ti K Initial temperature Tsat K Saturation temperature T∞ K Bulk temperature V m3 Volume 3 Vaf m Volume of water above fuel assemblies Greek Symbols α m2s−1 Thermal diffusivity β - Dimensionless structure coefficient λ Wm−1 Thermal conductivity µ cm2g−1 Gamma attenuation coefficient ν m2s−1 kinematic viscosity ρ kgm−3 Density Subscripts af Above fuel i Initial op Operation sat Saturation vap Vaporization vii viii Acknowledgements CONTENTS Abstract iii Acknowledgements v 1 Introduction 1 1.1 Background . .1 1.2 Thesis objectives . .2 2 March 2011 events at Fukushima Dai-ichi 3 2.1 The Natural Disaster . .3 2.2 Event sequence at Fukushima Dai-ichi . .4 3 Spent nuclear fuel pools, spent fuel and Decay heat 7 3.1 Spent Nuclear Fuel Pools . .7 3.2 Spent Nuclear Fuel . .8 3.2.1 Radiotoxicity . .8 3.3 Boil off times . 10 3.3.1 Boiling off . 10 3.3.2 Governing equations . 11 3.4 Results . 11 3.4.1 Case 1 . 12 3.4.2 Case 2 . 12 3.4.3 Case 3 . 12 3.5 Conclusions . 12 4 The possibility of air cooled passive safety systems 15 4.1 Design requirements . 15 4.2 Design Suggestion . 15 4.2.1 Copper wall design . 16 4.2.2 Wall strength and thickness . 16 4.3 Theory of heat transfer and governing equations . 18 ix x CONTENTS 4.3.1 Convection . 18 4.3.2 Conduction . 19 4.3.3 Heat transfer . 19 4.3.4 Air duct mass flow . 20 4.4 Results and feasibility . 20 4.4.1 Structual Integrity of the wall . 20 4.4.2 Heat transfer . 20 4.4.3 Verification with OpenFoam . 22 4.5 Conclusions . 22 5 Alternative design suggestions and emergency prepardness 25 5.1 Closed System . 25 5.2 Prolonging Designs . 25 5.2.1 Larger pools . 25 5.2.2 Implementing other types of existing passive cooling systems . 25 5.2.3 Enclosed pool . 26 5.2.4 Multiple pool heat sink . 26 5.2.5 Lessons from Fukushima Dai-ichi . 26 6 Discussion and Conclusion 27 Appendix A Verification with openFOAM 31 A.1 OpenFoam framewrok . 31 A.2 CFD.................................................. 31 A.2.1 Boussinesq approximation . 32 A.2.2 Newtonian Fluid . 32 A.2.3 Mesh . 32 A.2.4 Boundary conditions . 33 A.2.5 Accuracy of the results . 33 A.3 Post processing . 34 Appendix B Heat loss calculations 35 Appendix C Boil off times 37 Appendix D Passive safety system calculations 39 Appendix E OpenFoam Mesh 41 * LIST OF FIGURES 2.1 Picture showing the epicenter location of Japan March 2011 earthquake. .3 2.2 Picture showing units 1,2,3, and 4 starting from the right. .4 3.1 Total radiotoxic inventory in spent nuclear fuel as a funciton of time[13]. .9 3.2 The time dependency of decay heat along with some of the largest contributing isotopes[13]. .9 3.3 Thermal power after shut down for different burnup times top.................. 10 4.1 Passive safety system that was investigated. 16 4.2 Passive safety system that was investigated. 17 4.3 Copper wall design. 17 4.4 Stress distribution in copper wall. 21 4.5 Temperature distribution through copper wall [3] . 21 4.6 Pressure distribution. 22 4.7 Temperature distribution. 23 4.8 Flow velocities. 23 5.1 Alternative safety system design . 26 A.1 The data for the physical properties were extracted form the red line at the top of the duct. 34 xi xii List of Figures LIST OF TABLES 3.1 Relative power of fuel for different burnup times given in %. 10 3.2 Variables used for boil-off calculations. 12 3.3 Case 1 results. 12 3.4 Case 2 results. 12 3.5 Case 3 results. 12 3.6 Case 3 corrected results. 13 4.1 Temperature distribution for a full core unloading, case 1. 21 A.1 OpenFoam bondary conditions . 33 xiii xiv List of Tables CHAPTER 1 INTRODUCTION 1.1 Background The events that occurred in Japan in March of 2011 showed us that there might be parts of the nuclear industry that has a lack of proper safety margins. The nuclear reactors that managed to withstand such a powerful earthquake and an unprecedented tsunami belongs to the second generation of reactors that were built in the 1970’s. This freak of nature catastrophe had a very low probability of occurring but came very close to having disastrous consequences. Even though the design of these reactors belong to the history books there are still some similarities in the design between the oldest generation of reactors and the modern reactors that are being built and designed today, generation three and generation three plus. The Three Mile Island(TMI) accident in 1979 was a milestone withinin the nuclear industry that will not be forgotten. It showed us the importance of safety systems and control systems in a nuclear power plant. Maybe most importantly it showed us that the human factor can play a vital role in safety. Attention was given both to the possible direct actions of a single human being but also the behavior of an organization. Modern reactors incorporate safety systems that can even counter faulty human behavior. Even though TMI did not cause any dangerous release of radiotoxic material, it suffered a severe meltdown from a to that day unpredicted sequence of events. The mindset at that time was that large break.
Details
-
File Typepdf
-
Upload Time-
-
Content LanguagesEnglish
-
Upload UserAnonymous/Not logged-in
-
File Pages58 Page
-
File Size-