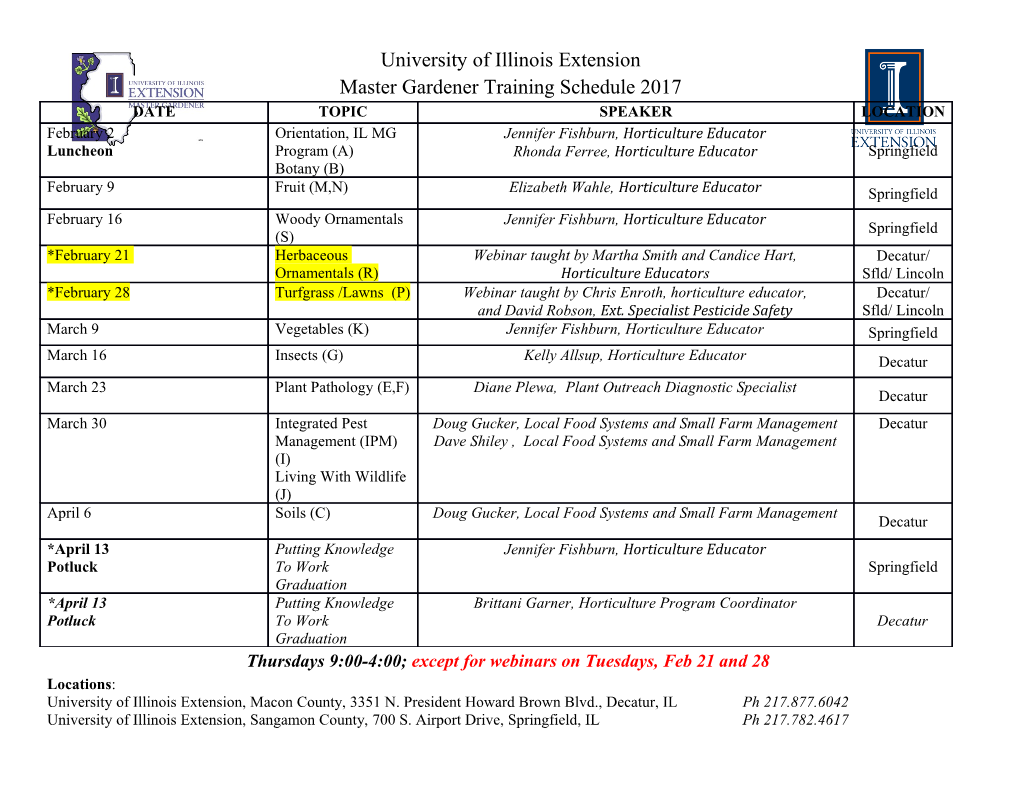
Stellar Atmosphere Volume 푉= (Area) (length) Collision = cross section 휎 ⋅ ℓ = 휎 퓋푡 Relative speed 퓋 Number of particles 푁 Number density 푛 = 푁Τ푉 # of collisions = # of (other) particles in the volume = 푁 = 푛 휎 퓋 t # of collisions per unit time = 푁 Τ푡 = 푛 휎 퓋 Time between 2 consecutive collisions (푁=1) (mean-free time), 푡col = 1 Τ (푛 휎 퓋) Distance between 2 consecutive collisions (푁=1) (mean-free path), ℓcol = 퓋푡col = 1 Τ (푛 휎 ) In general “encounters” between particles, or between a particle and a photon. The “cross section” is the key. 2 Opacity −1 ′ 2 −1 −3 휅 cm = 휅 휌 cm g ⋅ g cm = 푛푖 휎푖 푖 Recall that 휅 푑푠 is the optical depth If 휅휈 is frequency independent 휅 gray atmosphere Usually an average opacity is used 휅휈 퐵휈 푑휈 Planck opacity average of frequency 휅P = 퐵 푑휈 dependent opacity weighted by Planck function 휈 Svein Rosseland −1 Rosseland opacity weighted 휅휈 푢휈 푑휈 by 푇 derivative, averaging 1Τ휅휈 1Τ휅R = 푢휈 푑휈 푢 휈, 푇 = 휕퐵휈 푇 Τ휕푇 3 푎+푏+푐 Arithmetic average, , e.g., 1 + 4 + 4 Τ3 = 3 3 Geometric average, 3 푎 ∙ 푏 ∙ 푐, e.g., 3 1 × 4 × 4 ≈ 2.52 1Τ푎 + 1Τ푏 + 1Τ푐 −1 Harmonic average, one of the Pythagorean means, , 3 1Τ1 + 1Τ4 + 1Τ4 −1 e.g., = 2 3 4 Bound-bound absorption Excitation of an electron of an atom to a higher energy state by the absorption of a photon. The excited atom then will be de-excited spontaneously, emitting a photon, or by collision with another particle. Bound-free absorption Photoionization of an electron from an atom (ion) by the absorption of a photon. The inverse process is radiative recombination. 5 • Free-free absorption Transition of a free electron to a higher energy state, via interaction of a nucleus or ion, by the absorption of a photon. The inverse process is bremsstrahlung. • Electron scattering Scattering of a photon by a free electron, also known as Thomson (common in stellar interior) or Compton (if relativistic) scattering. • H− absorption Important when < 104 K, i.e., dominant in the outer layer of low-mass stars (such as the Sun) Hydrogen anion (or protide) Opposite is H cation (or hydron or just proton) 6 • Bound-bound, bound-free, and free-free opacities are collectively called Kramers opacity, named after the Dutch physicist H. A. Kramers (1894-1952). • All have similar dependence 휅 ∝ 휌 푇−3.5. • Kramers opacity is the main source of opacity in gases of 4 6 temperature 10 ~10 K, i.e., in the interior of stars up to ~ 1 M⊙. • In a star much more massive, the electron scattering process dominates the opacity, and the Kramers opacity is important only in the surface layer. Kramers opacity 휅 ≈ 4 × 1025 1 + 푋 푍 + 0.001 휌 푇−3.5 [cm2g−1] 퐾푟 7 X=0.70 Z=0.02 Rosseland mean opacity Data from Iglesias & Rogers (1996) 8 For Thomson scattering, 2 8휋 푟푒 2 −1 휅휈 = = 0.20 1 + 푋 [cm g ] 3 휇푒푚e is frequency independent, so is the Rosseland mean. 2 −1 휅푒푠 = 0.20 1 + 푋 [cm g ] Here 푟푒 is the electron classical (charge; Lorentz) radius, 푋 is the H mass fraction, and 휇푒 = 2Τ(1 + 푋) 푒2 푟 = = 2.82 × 10−15 [m]; experimentally 푟 < 10−18 [m] 푒 푚푐2 푒 −25 2 Classical electron cross section, 휎T = 6.65 × 10 [cm ] = 0.665 barns 9 10 11 Bowers & Deeming12 16 17 Absorption and Emission by Gas Hydrogen as an example … 18 QM versus classical physics Action [energy time] or (momentum distance] is quantized in unit of ℏ Heisenberg’s uncertainty principle, 휎푥 휎푝 ≥ ℏΤ2 Pauli exclusion principle Harwit 19 Lowest state of H, 푝2 푟2 ≈ ∆푝2 ∆푟2 ≈ ℏ2 Virial theorem, 2ℰ퐾 + ℰ푝 = 0 Lowest (ground state) energy 1 1 푍푒2 1 푝2 1 ℏ2 ℰ = − 퐸 = − = − ≈ − 1 2 푝 2 푟 2 휇 2휇 푟2 휇: reduced mass 푍푒2 ℏ2 ℏ2 = ⇒ 푟 = (퐁퐨퐡퐫’퐬 퐫퐚퐝퐢퐮퐬) 푟 휇푟2 휇푍푒2 1 푍푒2휇푍푒2 1 푍2휇푒4 ℰ = − = − 1 2 ℏ2 2 ℏ2 −9 For H, 푍 = 1, ℰ1 = −13.6 eV, 푟 ≈ 5.3 × 10 [cm] = 0.53 Å 20 ℎ ℎ de Broglie matter wavelength, 휆 = = 푝 푚푣 푍푒2 Virial theorem (classical uniform circular motion), 푚푣2 = 푟 Standing waves, 2휋 푟 = 푛휆 ℏ2 For the ground state, 푛 = 1, 푟 = 푚푍푒2 21 Virial theorem relation between (the time average of) the total kinetic energy and the total potential energy of a system in equilibrium Equation of motion (in the Lagragian form) 푑2푟Ԧ 휚 = 푓Ԧ − 훻푃 …… (1) 푑푡2 푑2푟Ԧ In hydrostatic equilibrium, = 0, so 푓Ԧ = 훻P, and assuming 푑푡2 spherical symmetry and the force being self-gravitation 푑푃 퐺 푚 푟 휚 푟 = − (Hydrostatic equilibrium) 푑푟 푟2 푟 2 (and 푚 푟 = 0 4휋푟 휚 푑푟 (mass continuity/distribution 22 푑2푟Ԧ 휚 = 푓Ԧ − 훻푃 푑푡2 Take the vector dot of 푟Ԧ of (1), divide by 휚, define 푭 = 풇Τ휚 (force per unit mass, and then integrate, using the boldface for vectors 풅ퟐ풓 푑푚 ( 푑푚 풓 ⋅ = 풓 ⋅ 푭 푑푚 − 풓 ⋅ 훻푃 …… (2 풅풕ퟐ 휚 푑 푑풓 푑2풓 푑풓 2 1 푑2 Given 풓 ⋅ = 풓 ⋅ + = 풓2 푑푡 푑푡 푑푡2 푑푡 2 푑푡2 풅ퟐ풓 1 푑2 푑풓 2 So, 푑푚 풓 ⋅ = 풓2 푑푚 − 푑푚 풅풕ퟐ 2 푑푡2 푑푡 1 푑2퐼 퐼: moment of inertia = − 2ℰ 2 푑푡2 kin ℰkin: kinetic energy 23 Because 푑푚 = 휚 푑푉, the last term in (2), 푑푚 풓 ⋅ 훻푃 = 풓 ⋅ 훻푃 푑푉 = 훻 ∙ 풓 ∙ 푷 푑푉 − 3 푃 푑푉 휚 Note 풓 ∙ 푷 ∙ 푑푺 − 3 푃 푑푉 = න 훻 풓푃 = 훻 ⋅ 풓 푃 + 풓 ⋅ 훻푃 훻 ⋅ 풓 = 3 Assuming spherical symmetry, Gauss’s theorem volume 3 integral of the divergence to 4휋푅 푃푠 − 3 푃 푑푉 surface integral = 24 Putting together, we have 1 푑2퐼 = 2ℰ + 3 න 푃 푑푉 + න 풓 ⋅ 푭 푑푚 − ර 푃풓 ⋅ 푑푺 2 푑푡2 kin where 풓 ⋅ 푭 (work) is virial; or 1 푑2퐼 = 2 ℰ + 3 න 푃 푑푉 + ℰ − 4휋푅3푃 2 푑푡2 kinetic potential external For stars, under hydrostatic equilibrium and if 푃ext = 0, 2 ℰk + ℰp = 0 25 1 푑2퐼 LHS = 0 stable = 2 ℰ + ℰ 2 푑푡2 k p LHS < 0 collapsing LHS > 0 expanding 퓔퐤: a variety of kinetic energies 퓔퐩: a variety of potential energies Kinetic energy of molecules Gravitation Bulk motion of clouds Magnetic field Rotation Electrical field … … Note ℰtotal = ℰ푘 + ℰ푝, governs if the system is bound (ℰtotal < 0) For stars, mostly ℰ푝 = Ω (gravitational energy; negative) 26 For higher energy states, 푝푛 푟푛 = 푛ℏ 2 2 2 2 4 푝푛 푛 ℏ 푍 휇 푒 ℰ푛 = − ≈ − 2 = − 2 2 2휇 2휇푟푛 2푛 ℏ For the 푛-th radial state, the phase space volume is 2 2 4휋푝푛 Δ푝푛 4휋푟푛 Δ푟푛 , # of possible states with principle quantum number 푛 Total phase space volume 16휋2푛2ℏ3 = = ∝ 푛2 volume of unit cell ℏ3 The electron spin is either parallel or anti-parallel to that of the nucleus, so the n-th state has 2푛2 different substates, all having the same energy. 27 28 푍2휇 푒4 Note that ℰ ∝ 휇 ℰ = − 푛 푛 2푛2ℏ2 푚푒푚푝 푚푒 For normal H, 휇퐻 = = ≈ 푚푒 1 − 푚푒Τ푚푝 푚푒+푚푝 1+푚푒Τ푚푝 푚푒푚퐷 2푚푒푚푝 For deuteron, 휇퐷 = = ≈ 푚푒 1 − 푚푒Τ2푚푝 > 휇퐻 푚푒+푚퐷 푚푒+2푚푝 So the D lines are 1.5 Å shorter in wavelengths 2 Note also that ℰ푛 ∝ 푍 , so for He II (푍 = 2, with 1 e−) , 푍2 is 4 times larger, and with a different 휇. https://archive.stsci.edu/fuse/scisumm/sci_d2h.html29 푛 Grotrian diagram Lang Gray & Corbally30 31 • For the ground state, the orbital angular momentum is ℓ = 0. The total spin angular momentum is 퐹 = 0 (spin opposite) or 퐹 = 1 (spin parallel) Hyperfine splitting • For 푛 = 2, ℓ =1, and with spin, a total angular momentum of ℓ ℓ + 1 ℏ2 =2ℏ2 3 substates, ℏ, 0, −ℏ, 푚 = 1, 0, −1 (magnetic quantum number) Fine structure, ∆ℰ very small, ~10−5 Ev But if there is an external B field Zeeman splitting 32 Free-free 퐇+ Free-free or free-bound to any level Cascading down emission of photons of different energies − 퐇 Stars: ample supplies of free 푒− from Na, 퐻 + 푒− ⟶ 퐻− + ℎ휈 Ca, Mg, … with low-ionization potentials He atom similar, with the second 푒− weakly bound, shielded by the first 푒− − ℰbinding H = 0.75 eV, only 1 bound state; transitions continuum Absorption by H− immediately followed by reemission − The sunlight we see mostly is due to continuum transitions by 퐻 33 퐇ퟐ • Main constituent of cold clouds, not important in stars, except in the coolest substellar objects (brown dwarfs or planetary- mass objects) • Lacking a permanent electric dipole moment, so very difficult to detect. A rotationally excited molecule would radiate through a relatively slow electric quadrupole transition. • Only detected in a hot medium, where stellar radiation or stellar wind excites vibrational and electronic states which then decay relatively quickly. Zero electric dipole moment 34 Stahler & Palla 35 CO molecules • Simple and abundant, in gaseous or solid form • Strong ℰbinding =11.1 eV self-shielding against UV field • with a permanent electric dipole moment; radiating strongly at radio frequencies. • 12C16O easiest to detect; isotopes 13C16O, 12C18O, 12C17O, 13C18O also useful • Low critical density for excitation CO used to study large- scale distribution of molecules, as a tracer of H2 in dense clouds • 12C16O almost always optically thick; same line from other rare isotopes usually not estimate of column density (total mass) 6 of molecular gas 푁퐻= 10 푁13 퐶푂 36 2.6 mm = 115 GHz Only 5 K above the ground level … can be excited by collisions with ambient molecules or CMB photons Stahler & Palla 37 CO bandheads in the Becklin-Neugebauer (BN) object, an IR-emitting, embedded, massive (~7 M⊙) protostar Gaballe & Persson (1987) Stahler & Palla38 Stahler & Palla 39 Molecules in stars Stellar matter largely gas or plasma.
Details
-
File Typepdf
-
Upload Time-
-
Content LanguagesEnglish
-
Upload UserAnonymous/Not logged-in
-
File Pages76 Page
-
File Size-