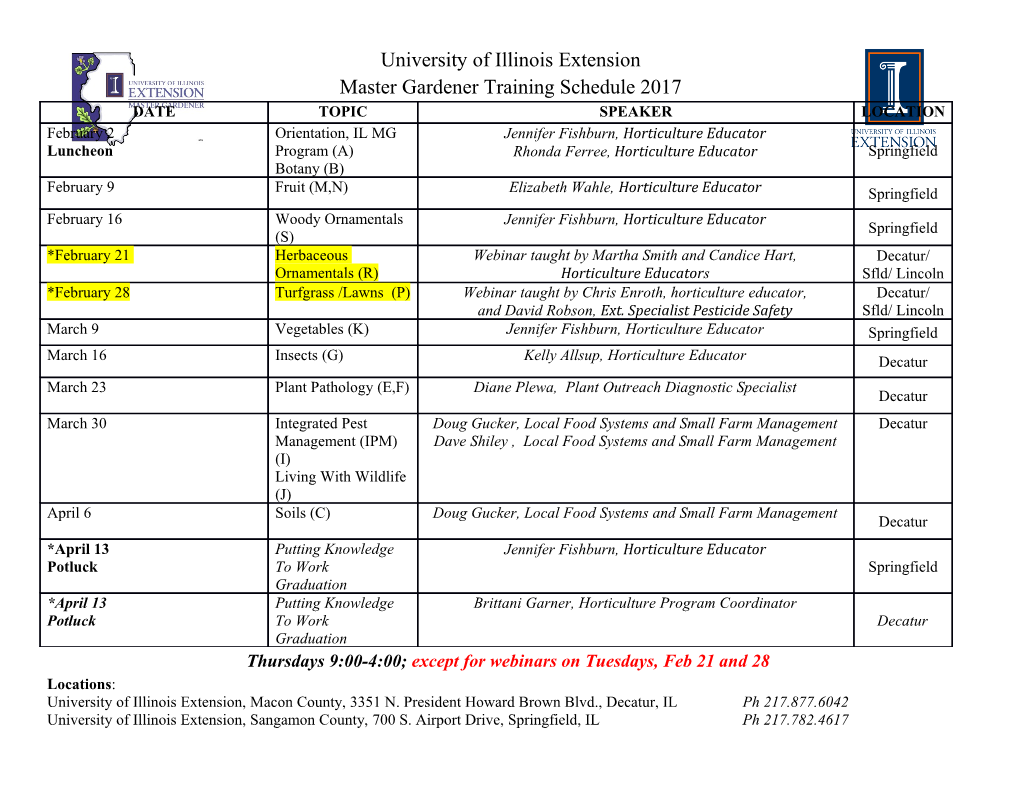
The effect of N-terminal mutations on the thermostability of VPR, a cold active subtilisin-like serine protease Árni Thorlacius FacultyFaculty of of Physical Physical Sciences Sciences UniversityUniversity of of Iceland Iceland 20192019 THE EFFECT OF N-TERMINAL MUTATIONS ON THE THERMOSTABILITY OF VPR, A COLD ACTIVE SUBTILISIN-LIKE SERINE PROTEASE Árni Thorlacius 15 ECTS thesis submitted in partial fulfillment of a Baccalaureus Scientiarum degree in Biochemistry Advisor Prof. Magnús Már Kristjánsson Co-advisor Kristinn Ragnar Óskarsson, M.Sc. Faculty of Physical Sciences School of Engineering and Natural Sciences University of Iceland Reykjavik, May 2019 The effect of N-terminal mutations on the thermostability of VPR, a cold active subtilisin- like serine protease 15 ECTS thesis submitted in partial fulfillment of a B.Sc. degree in Biochemistry Copyright © 2019 Árni Thorlacius All rights reserved Faculty of Physical Sciences School of Engineering and Natural Sciences University of Iceland Dunhagi 5 107, Reykjavik, Reykjavik Iceland Telephone: 525 4000 Bibliographic information: Árni Thorlacius, 2019, The effect of N-terminal mutations on the thermostability of VPR, a cold active subtilisin-like serine protease, B.Sc. thesis, Faculty of Physical Sciences, University of Iceland. Printing: Háskólaprent, Fálkagata 2, 107 Reykjavík Reykjavik, Iceland, May 2019 Útdráttur Rannsóknarverkefnið byggir á fyrri rannsóknum varðandi byggingareinkenni sem ákvarða hitastigsaðlögun í mismunandi próteinum, þar sem borin voru saman tvö samstofna ensím: C-enda stytt afbrigði af kuldaaðlöguðu VPR (VPR∆C) úr kuldakærri Vibrio örveru, og hitastöðugt aqualysin I (AQUI) úr hitakæru örverunni Thermus aquaticus. Stökkbreytingar við N-endann hafa áhrif á víxlverkanir milli N-enda lykkjunnar og megin- byggingar þessara ensíma og þ.a.l. á hitastöðugleika þeirra. Tvöfalda prólín stökk- breytingin N3P/I5P jók hitastöðugleika VPR meðan að W6F stökkbreytingin lækkaði hann. Tilgangur þessa verkefnis var að tjá allar þrjár stökkbreytingarnar samtímis í einu afbrigði, VPR∆C/N3P/I5P/W6F, til að skoða áhrif þeirra á hitastöðugleika og hraðafræðilega eiginleika, þ.e.a.s. að athuga hvort jákvæð áhrif N3P/I5P á hitastöðug- leika VPR trompi neikvæð áhrif W6F. N3P/I5P/W6F afbrigðið sýndi lægri hvötunarvirkni en villigerðarensímið. Hún var einnig lægri en hvötunarvirkni N3P/I5P og W6F afbrigð- anna. Hitastöðugleikamælingar á N3P/I5P/W6F afbrigðinu gáfu lægri Tm og T50% gildi ◦ ◦ en fyrir villigerðina, ∼12-13 C lægra og ∼3 C lægra, í hvoru tilfelli. Tm og T50% voru lægri en hjá N3P/I5P afbrigðinu, Tm var einnig lægra en hjá W6F afbrigðinu. T50% ◦ var hins vegar hærra en hjá W6F, og það sem meira er, ∼1 C hærra en Tm sem hefur aldrei mælst hingað til í öðru afbrigði VPR. Byggingin umhverfis hvarfstöðina gæti verið stöðugri en annars staðar í ensíminu. DSC mæling á VPR∆C/N3P/I5P/W6F renndi stoðum undir þessa ályktun, en hún gaf til kynna að a.m.k. einn varmafræðilegur atburður á sér stað við hærra hitastig en hjá hinum. Jákvæð áhrif N3P/I5P á hitastöðugleika VPR trompa því ekki neikvæð áhrif W6F. Abstract This research project is a part of a larger project aimed at determining the structural basis for temperature adaptations in proteins by studying two subtilisin-like serine proteases that are structurally homologous: A C-terminal truncated form of the cold-active VPR (VPR∆C), from a psychrophilic Vibrio species, and the thermostable aqualysin I (AQUI), from the thermophile Thermus aquaticus. Substitutions close to the N-terminus affect interactions between the N-terminal loop and the main body of these enzymes, which in turn affects their thermostability. The double proline substitution N3P/I5P increased the thermostability of VPR but the W6F substitution decreased it. The purpose of this project was to express all three mutations simultaneously in one variant, VPR∆C/N3P /I5P/W6F, and observe their effect on both thermostability and kinetic parameters, i.e. to see whether the stabilizing effect of N3P/I5P could overcome the destabilizing effect of W6F. The N3P/I5P/W6F variant displayed much lower catalytic activity than the wild type enzyme and both N3P/I5P and W6F variants. Thermostability measurements ◦ generated lower Tm and T50% values than for the wild type enzyme, ∼12-13 C lower and ◦ ∼3 C lower, respectively. Both Tm and T50% were lower than those of the N3P/I5P variant, Tm was also lower than that of the W6F variant. The T50% was however ◦ higher than that of the W6F variant, and more interestingly, ∼1 C higher than Tm which has never been observed before in any variant of VPR. This could be due to the scaffold around the enzymes active site being more stable than the surrounding structures. Indeed, a DSC measurment of VPR∆C/N3P/I5P/W6F revealed at least one thermal event occuring at a higher temperature than the rest. The stabilizing effect of N3P/I5P was apparently insufficient to overcome the destabilizing effect of W6F. Contents List of Figures ix List of Tables xiii 1 Introduction1 1.1 Protein stability . .2 1.2 Protein adaptations to temperature . .5 1.2.1 Thermodynamic stability . .5 1.2.2 Flexibility . .7 1.3 Proteases . 11 1.3.1 Serine proteases . 12 1.3.2 Subtilisin-like serine proteases . 14 1.3.3 VPR . 15 1.4 The aim of the project . 20 2 Materials and methods 21 2.1 Protein purification . 21 2.1.1 Purification of VPR∆C and VPR∆C/N3P/I5P/W6F . 21 2.1.2 Zaman-Verwilghen protein quantitation . 22 2.1.3 SDS-PAGE . 22 2.2 Enzymatic activity assays . 24 2.3 Michaelis-Menten kinetics . 24 2.4 Stability measurements . 26 2.4.1 Melting point (Tm) determination . 26 2.4.2 Thermal inactivation (T50%) measurements . 27 2.4.3 Differential scanning calorimetry (DSC) . 28 vii Contents 3 Results and discussions 29 3.1 Purification . 29 3.1.1 SDS-PAGE . 31 3.2 Michaelis-Menten kinetics . 32 3.3 Stability measurements . 33 4 Conclusions 37 5 References 39 viii List of Figures 1.1 The trade-off relationship between thermostability and catalytic activity at low temperatures in homologous enzymes. Thermophilic enzymes display high thermostability but low catalytic activity at low temperatures and psychrophilic enzymes display opposite qualities. The area shaded blue is where natural enzymes are located, and to its left is the area where the minimal activity and stability requirements for practical enzymes are not met, which is colored pink. Enzymes that would be located in the upper-right corner, i.e. highly thermostable and active at low temperatures, can be developed in the laboratory, but are generally not found in nature (15). ...........................3 1.2 (a) Diagram linking protein-thermodynamic stability (∆GU) and folding/ unfolding rate constants (kF/kU), in a two-state model, where unfolding z z is reversible. TS denotes the high-energy transition state and ∆GF/∆GU the energy required to transition between the two states. (b) The three thermodynamic models used to explain increased thermodynamic stability in thermostable enzymes, compared to a mesophilic homolog (black solid line) (8)...................................6 1.3 Diagram of the Maxwell-Boltzmann distributions of molecules at different temperatures in Kelvin along with the activation energies necessary for two different reactions. At 173 K the portion of molecules that have enough kinetic energy that is required for either reaction is negligible. At 273 K a small portion of molecules has enough energy for the first reaction (dark grey). At 373 K some molecules finally have the kinetic energy that is needed for the second reaction (6). .8 ix LIST OF FIGURES 1.4 Diagram showing the different energy landscapes for psychro- and thermo- philic enzymes. The wider the funnel the more unfolded conformations there are, which are high in Gibbs free energy. The longer the funnel the more stable the native states of the enzymes compared to their unfolded states. The valleys in the bottom house stable conformations and the ridges in between them indicate the energy barrier the enzymes need to overcome to switch between stable conformations (7). .9 1.5 Schematic representation of the mechanism for serine proteases. Number- ing of residues corresponds to that of chymotrypsin-like proteases (26). 13 1.6 Diagram of a substrate (P2’-P4) bound to the substrate binding sites (S2’-S4) of a subtilisin-like serine protease. Hydrogen bonds between substrate and protein are shown as dotted lines. Enzyme numbering is that of subtilisin BPN’. The catalytic residues D32, H64, and S221, and the oxyanion hole residue N155 have been labeled (27). 15 1.7 Schematic representation showing the maturation of VPR and AQUI (30). 16 1.8 Three-dimensional model of VPR from a psychrotrophic Vibrio species (PDB ID: 1SH7), made using the program UCSF Chimera. Calcium ions are colored green, disulfide bonds yellow, and the N-terminal region orange. Residues of the catalytic triad (Asp37, His70 and Ser220), the stabilizing Trp6 residue, and disulfide bonds have been labeled, along with residues located at the site of an important salt bridge in AQUI (Asn15 and Lys257 in VPR). 17 1.9 Topology diagram of the structure of VPR (34). 18 1.10 Close-up of a three-dimensional model of VPR (light blue, PDB ID: 1SH7) superimposed on to a three-dimensional model of AQUI (orange, PDB ID: 4DZT), made using the program UCSF Chimera, which shows some of the differences between the amino acid composition of their N-termini. 19 x LIST OF FIGURES 3.1 SDS-PAGE of purified samples of VPR∆C and VPR∆C/N3P/I5P/W6F. ™ Lane 1: PageRuler Prestained Protein Ladder. Lane 2: VPR∆C (Table 3.1). Lane 3: VPR∆C/N3P/I5P/W6F (Table 3.2). The size of the proteins, in the protein ladder, is marked in kDa. 31 3.2 Example of two kinetic assay data sets (black dots) for VPR∆C/N3P/ I5P/W6F, along with error bars, fitted to Michaelis-Menten equations (red and blue lines).
Details
-
File Typepdf
-
Upload Time-
-
Content LanguagesEnglish
-
Upload UserAnonymous/Not logged-in
-
File Pages59 Page
-
File Size-