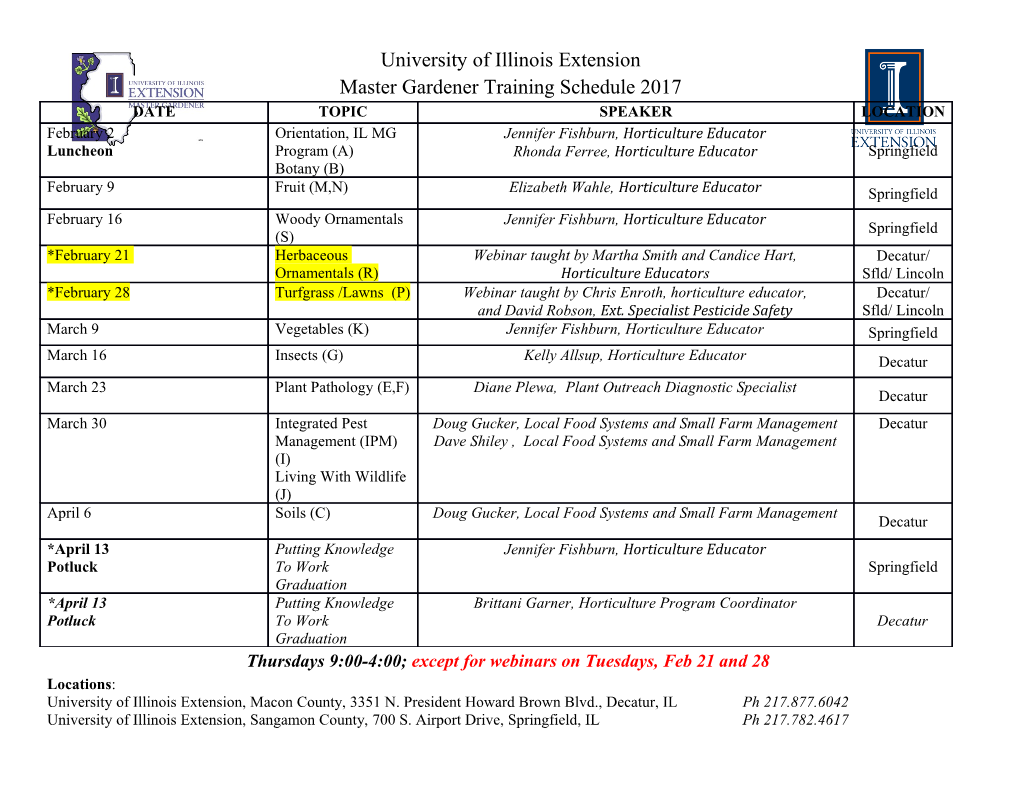
Header for SPIE use Primary aberration coefficients for axial gradient-index lenses Florian Bociort Optics Research Group, Department of Applied Physics Delft University of Technology, The Netherlands* ABSTRACT As for homogeneous lenses, for axial gradients the analysis of the Seidel and chromatic aberration coefficients can be very useful in lens design. However, at present few commercial optical design programs list the Seidel aberrations of GRIN lenses and none of them lists the chromatic aberrations. In order to facilitate the computer implementation of the chromatic aberrations of axial GRIN lenses a new mathematical derivation for the axial and lateral color coefficients is presented. Also, new qualitative insight into the properties of axial GRIN lenses is obtained by introducing the thin-lens approximation in the aberration expressions. Within the domain of validity of this approximation, the primary aberrations of an axial GRIN lens are equivalent to those of a pair of homogeneous aspherical lenses in contact, having a common plane surface and having refractive indices and Abbe numbers equal to the corresponding axial GRIN values at the two end surfaces. Keywords: lens design, gradient-index optics, aberrations, geometrical optics 1. INTRODUCTION The interest in gradient-index (GRIN) optics has increased recently as a result of substantial progress in the manufacturing technology of GRIN components. Major progress has been achieved especially for axial gradients (i.e. glasses for which the refractive index is a function of the coordinate along the symmetry axis) 1,2. It is well known that spherical surfaces of axial GRIN lenses have aberration correction capabilities that are nearly equivalent to those of aspheric homogeneous lenses, and therefore axial gradients are used basically as asphere replacements. However, the effect on the monochromatic and chro- matic aberrations of the ray propagation through the axial GRIN medium between the surfaces is more difficult to be ana- lyzed. It is convenient for this purpose to examine the Seidel and chromatic paraxial aberration coefficients (i.e. the primary aberrations) of the axial GRIN lens: Unlike propagation in homogeneous media, ray propagation through gradients gener- ates so-called transfer contributions to the primary aberrations. For instance, the values of the primary aberrations can be used to investigate the sensitivity of the aberrations when the gradient material is changed. Analytic expressions of the primary aberrations of axial GRIN lenses have been derived some time ago by Sands3,4,5,6. How- ever, Sands’ complex mathematical derivation is quite a challenge for many interested readers. At the time of this writing only one of the major commercial optical design programs (CODE V) lists the Seidel aberrations of gradients and none of them lists the chromatic aberrations. The present paper has two objectives. First, in order to facilitate the computer implementation of the chromatic aberrations of axial GRIN lenses a new mathematical derivation for the transfer contributions axial and lateral color coefficients will be presented. The derivation described in Section 4 is based on the method first developed by this author for the aberration coefficients of radial GRIN lenses7. The results are equivalent with those of Sands, but the derivation itself is perhaps sim- pler and certain intermediate results can also be used to check the accuracy of the computer implementation of the formulas and to avoid possible implementation errors. As a prerequisite, ray tracing will be reviewed in Sec. 2. The formulas that are necessary for the computation of the primary aberrations are summarized in Sec.3. The second objective of this paper is to gain a better qualitative understanding of the effect of the transfer contributions for all primary aberrations of axial gradients. For this purpose, in Section 5 the thin-lens approximation will be introduced in * Address: Lorentzweg 1, 2628 CJ Delft, E-mail:[email protected], Permanent address: National Institute for Laser, Plasma and Radiation Physics, Department of Lasers, P.O. Box MG-36, 76900 Bucharest, Romania the aberration expressions. It will be shown that, within the domain of validity of this approximation, the primary aberra- tions of an axial GRIN lens are equivalent to those of a pair of homogeneous aspherical lenses in contact, having a common plane surface and having refractive indices and Abbe numbers equal to the corresponding axial GRIN values at the two end surfaces. 2. RAY TRACING IN AXIAL GRADIENTS As for homogeneous lenses, the primary aberrations of axial gradients are computed by using paraxial ray-tracing data for the marginal and chief ray. Therefore, ray tracing in axial gradients will be briefly reviewed below. The path of a ray in an inhomogeneous medium is described by the well - known differential equation d ⎛ dR ⎞ ⎜n ⎟ = ∇n (1) ds ⎝ ds ⎠ where n(x,y,z) is the refractive index of the medium, R = (x,y,z) is the position vector and ds denotes the arc length between two neighboring points along the ray path, such that we have dsdxdyd2 =++2 2 z 2 . The vector n dR/ds is of length n and has as its components the three optical direction cosines ξ,η,ζ with respect to the axes x, y and z , respectively, which are related by ξ222++=η ζ n2 . In axial gradients, the refractive index changes only along the symmetry axis, n=n(z). Since ∂∂nx//== ∂∂ ny0 we have dξ //ds== dη ds 0 and it follows that in axial gradients the optical direction cosines with respect to x and y dx dy n ==ξ , n η (2) ds ds are invariant along the ray path, retaining the initial values ξ and η they had at the entrance in the medium. The third optical direction cosine dz n ==ζ nz2 () −ξ 2 −η 2 (3) ds is then simply a function of z . (We assume that the ray propagates from left to right and therefore choose the plus sign for the square root in Eq.(3).) For determining the ray path, note that Eqs. (2-3) yield dx ξ dy η ==, (4) dz ζ dz ζ The equations (4) can be integrated directly. Let x and y be the ray coordinates at the entrance in the medium and let x' and y' be the corresponding values after the ray travels a distance d in the medium. The solutions read xxA′ =+ξ ()ξ,ηη, yyA′ =+ ()ξ,η (5) where we have denoted d dz A()ξ,η = ∫ (6) 0 nz2 ()−−ξ 2 η 2 ω ==+16−1 ξ 2 η2 Introducing the abbreviations nz, Cξη we can write ω 1 1 3 (7) = =+ωωCξη +... 222 2 nz()−−ξ η 1 − Cξηω 2 Therefore we have 1 Andnd()ξ,η =+−−1 3 ()ξ 2 ++η 2 ... (8) 2 where we have denoted the average values of 1/n and 1/n3 by 1 d dz n −1 = ∫ (9) d 0 nz() and 1 d dz n −3 = ∫ (10) d 0 nz()3 In the paraxial approximation only the first term in the power series expansion (8) is retained, and Eqs. (5) become xxnd′ =+ −−1 ξ , yynd′ =+ 1 η (11) Note that the paraxial transfer equations for axial gradients are the same as for homogeneous media, excepting that the re- ciprocal of the homogeneous refractive index is replaced by its average value given by Eq. (9). The second term in Eq.(8) leads to a third-order term in Eqs.(5) and therefore the quantity (10) will enter in the expressions of the Seidel coefficients for transfer. For axial gradients, the refraction of paraxial rays at a surface is described by precisely the same equation as in the homoge- neous case. However, since the refractive index is variable within the lens, the value of the refractive index to be used is that at the surface vertex. Consider a rotationally - symmetric optical system consisting of homogeneous and gradient - index lenses. We define an τ τ arbitrary ray through the system by its normalized field coordinates ( x, y) in the object plane P, and by the aperture coor- σ σ dinates ( x, y) in the entrance pupil plane EP. Thus, if rEP is the radius of the entrance pupil and rP is the maximum object height, the Cartesian ray coordinates at the object plane and at the entrance pupil plane are given by ==ττ ==σσ xPPxPPryr, y , xEPryr EP x, EP EP y (12) At each surface, the position and direction of the ray are given by the x and y coordinates of its point of intersection with the surface and by the optical direction cosines ξ and η. A j B m i h u w O S C P Fig. 1 Ray parameters of the marginal ray OB and chief ray AP at the first surface of the system In the following sections we will need the position and direction of the ray only in the paraxial approximation. In this case it ξ η σ σ τ τ can be shown that at each surface of the system x,y, , are given by linear combinations of x, y, x, y and that the coeffi- cients are the height and slope of the paraxially traced marginal and chief rays at that surface xm=+τσ h, ξ =−− nwτσ nu xx0 x0 x . (13) =+τσ η =−− τ σ ymyy h, nw0 y nu0 y Here, the paraxial marginal and chief ray heights are denoted h and m and the corresponding marginal and chief ray slopes are denoted u and w. (See Fig.1.) The sign convention adopted for u and w is that their signs are the opposite of those of the corresponding direction cosines. (This is why in Eqs. (13) we have minus signs in the equations for ξ and η.) The refractive index at the vertex of the surface is denoted by n0.
Details
-
File Typepdf
-
Upload Time-
-
Content LanguagesEnglish
-
Upload UserAnonymous/Not logged-in
-
File Pages12 Page
-
File Size-