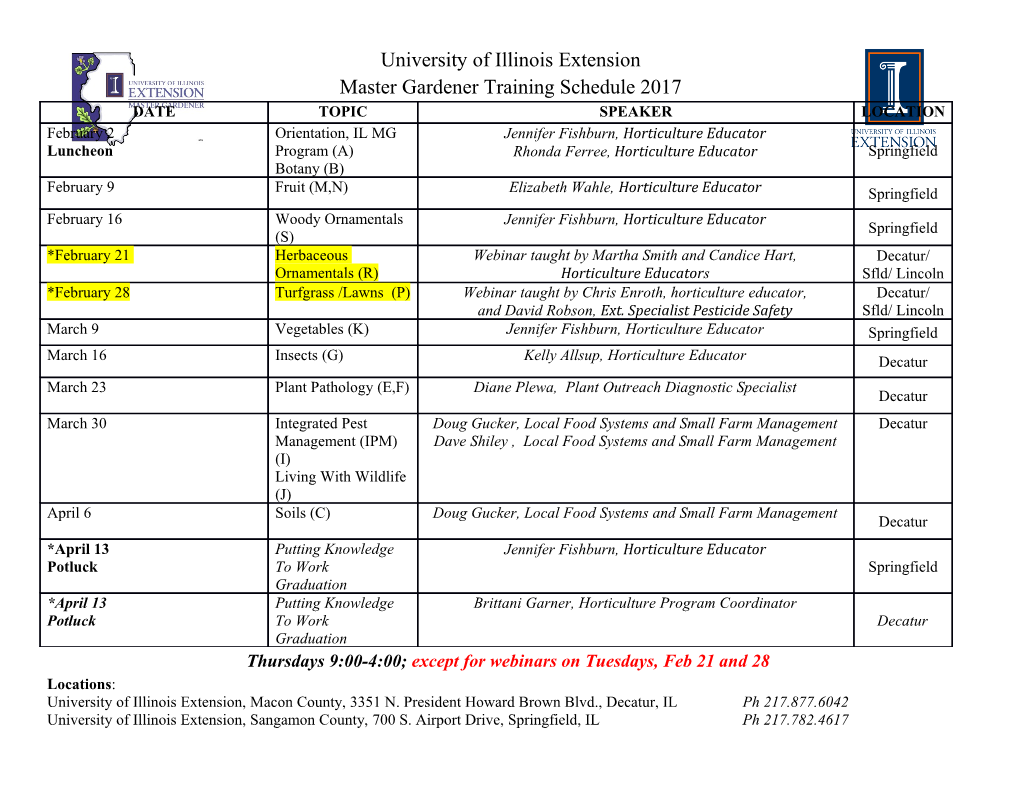
5 Early Differentiation and Core Formation: Processes and Timescales Francis Nimmo1 and Thorsten Kleine2 ABSTRACT The Earth’s core formed via a series of high‐energy collisions with already‐differentiated objects, likely resulting in several distinct magma ocean epochs. The cores of these impactors probably underwent only limited emulsification and moderate (~50%) isotopic re‐equilibration with the target mantle during the collision. Later impactors likely originated from more distant regions of the inner Solar System and were plausibly more volatile‐rich and more oxidized than earlier impactors. Short‐lived isotopes, especially the hafnium‐tungsten (Hf‐W) system, provide the strongest constraints on the timescale of accretion and core formation. These short‐ lived isotopes and dynamical models provide a mutually self‐consistent, albeit approximate, chronology. Terrestrial core formation took more than 30 Myr but less than about 200 Myr to complete. 5.1. IntrODUCTION did core formation take place? In the second half, we The Earth—and the other terrestrial planets—are focus on “when”; what evidence do we have for the differentiated bodies, a metallic core overlain by a sili­ timescales of melting and core formation? Although the cate mantle. But the nebula from which these planets main focus of this chapter is the Earth, the processes of ultimately formed originally consisted mainly of undif­ accretion and differentiation are general. As a result, ferentiated dust grains. The aim of this chapter is to considerable insight can be gained by looking at the evi­ review how and when the process of differentiation is dence from both meteorites and other terrestrial bodies, thought to have taken place. and we do so briefly in this chapter. In the first half of this chapter we focus on “how”; how The contents of this chapter are closely related to others did the planets ultimately grow, and by what mechanisms in this volume. In particular, the processes by which the elemental and stable isotopic composition of the core were established are examined in Chapter 6. We do not focus on silicate differentiation (mantle melting and crust 1Department Earth and Planetary Sciences, University of formation), which is treated in more detail in Chapter 8. California, Santa Cruz, California, USA Nor do we discuss the final addition of material to the 2Institut für Planetologie, Westfälische Wilhelms‐Universität mantle (the “late veneer”) after core formation effectively Münster, Münster, Germany ceased (see Chapter 4). The Early Earth: Accretion and Differentiation, Geophysical Monograph 212, First Edition. Edited by James Badro and Michael Walter. © 2015 American Geophysical Union. Published 2015 by John Wiley & Sons, Inc. 83 84 THE EARLY EARTH This chapter covers similar ground to various earlier on. This process slows once the local feeding zone is reviews. An old but still useful treatment of the physics of exhausted, and transitions into the more orderly third core formation is given by Stevenson [1990]; an accessible stage of oligarchic growth [Kokubo and Ida, 1998]. At a survey of the earliest Earth is by Zahnle et al. [2007]. A few Myr after Solar System formation, the region around comprehensive review with a similar scope to this one may 1AU will have contained tens of Moon‐ to Mars‐sized be found in Rubie et al. [2015]. “embryos” embedded within a cloud of surviving smaller planetesimals. At about the same time, early stellar activ­ 5.1.1. How Does Core Formation Occur? ity will have removed any remaining gas and dust not already incorporated into larger objects. The presence of In one sense, core formation is a very simple process. It gas is important because it can drive planetary migration. is energetically favorable for the densest components of a For instance, early migration of Jupiter and Saturn may planet (the metals) to sink to the center. However, this have sculpted the protoplanetary disk from which the redistribution of mass requires deformation of some kind terrestrial planets subsequently formed, potentially to occur. Neither metals nor silicates are particularly explaining the small mass of Mars [Walsh et al., 2011]. deformable at low temperatures, and as a result, core The final stage of terrestrial planet accretion involves formation can only occur at elevated temperatures growth via collisions with embryos; typical timescales for (Section 5.1.1.3). As we discuss in Section 5.1.1.2, the this process are tens of Myr [e.g., Agnor et al., 1999]. extent to which planetary bodies are heated as they grow From the point of view of the early Earth, this stage is the depends on the details of the accretion process. Our most important, because it involves the largest transfers current understanding of terrestrial planet accretion is of mass and energy (see below). Because of the relatively summarized in Section 5.1.1.1. small number of bodies involved, this process can be An important conclusion, at least for the Earth, is modeled relatively easily. Unfortunately, however, the that many of the bodies that formed the Earth were process is stochastic, and so there are many different themselves already differentiated. As a result, the idea of growth scenarios that could have yielded the early Earth. there being a single instant of core formation is too Figure 5.1 shows the typical growth history of an simple; in reality, the delivery of a metallic component to Earth‐like body (bold line), from the numerical simula­ the deep interior happened many times, and potentially tions of Raymond et al. [2006]. Growth occurs mainly by several different mechanisms. To the extent that it via half‐a‐dozen or so collisions with comparable‐sized implies a single event, “core formation” is thus a misnomer. objects; although there is a steady background mass accu­ We nonetheless continue to employ it as useful shorthand mulation via small impacts, the total mass fraction con­ for the processes by which metallic materials are trans­ tributed by this process is small. This kind of growth ported to depth. history can be crudely approximated by continuous growth at an exponentially‐decreasing rate (thin line). 5.1.1.1. Overview of Accretion in the Terrestrial Although this kind of analytical model is advantageous Planet Zone for modeling the isotopic effects of core formation (see The processes of planetary accretion and core growth Section 5.2.3 below), it does not capture the discontinu­ are inextricably linked. Planets grow via collisions, which ous, stochastic nature of the real accretion process. both deliver core material and produce conditions under Beyond the discontinuous, stochastic nature of Earth’s which differentiation is favored (see below). As a result, growth, there are two other relevant characteristics of we will begin with a brief overview of accretion. More accretion. First, the “feeding zone” from which the proto‐ detailed reviews may be found in Chambers [2010], Earth accretes material expands with time [O’Brien et al., Morbidelli et al. [2012], and Chapter 3. 2006, 2014; Bond et al., 2010]. As a result, material Conventionally, terrestrial planet accretion is thought delivered later in the accretion process tends to have to occur in four stages. The initial growth from micron‐ originated at greater distances from the Sun. This result is sized dust grains to kilometer‐sized bodies is not well potentially important for volatile content and partitioning understood, because of the tendency of meter‐scale bodies behavior of siderophile elements during core formation to break themselves apart. Nonetheless, this process must (see Section 5.2.3.3). Second, in reality only roughly one have occurred rapidly–in perhaps 103 years–to avoid loss in every two collisions actually result in the two bodies of the dust grains via gas drag. Kilometer‐scale bodies merging [Kokubo and Genda, 2010, Chambers, 2013]; are large enough to start perturbing their neighbors’ other collisions are either “hit‐and‐run” events [Asphaug orbits, at which point a second stage, that of runaway et al., 2006] or (more rarely) result in net mass loss (erosion) growth, ensues. In this stage, larger bodies are more effec­ of the target body. Hit‐and‐run events prolong the dura­ tive in focusing impacts than their smaller neighbors, and tion of the final stages of accretion. Erosive events are so grow more rapidly, becoming yet more effective and so important because they can change the bulk composition EARLY DIFFERENTIATION AND CORE FORMATION: PROCESSES AND TIMESCALES 85 1. 0 0.8 ) E M 0.6 Mass ( 0.4 Giant impact Dynamical model 0.2 Continuous growth (analytical) 0 20 40 60 80 100120 140 Time (Myr) Figure 5.1 Bold line shows a typical growth curve from an N‐body simulation [run2a of Raymond et al., 2006]; arrows indicate giant impacts. The thin line assumes growth at an exponentially decaying rate (equation 5.4) with a timescale τ of 10 Myr and a later final Moon‐forming impact. Exponential growth models with longer e‐folding time- scales have been used to reproduce the Hf‐W and U‐Pb isotope systematics of the Earth [Halliday, 2004; Rudge et al., 2010] under the assumption of incomplete re‐equilibration during impacts (Section 5.2.4.1). ME is one Earth mass. (and isotopic signature) of the target bodies [Dwyer et al., will be buried sufficiently deep that cooling via radiation 2015]; for instance, the Earth may have a non‐chondritic is ineffective, and as a result the body heats up. In the bulk composition (see Chapter 2) thanks to preferential simplest scenario, this effect results in an inverted impact removal of incompatible elements contained in temperature profile (later impactors deliver more energy), the crust [O’Neill and Palme, 2008]. We discuss this issue but for Earth‐sized bodies, this effect is likely to be further below.
Details
-
File Typepdf
-
Upload Time-
-
Content LanguagesEnglish
-
Upload UserAnonymous/Not logged-in
-
File Pages20 Page
-
File Size-