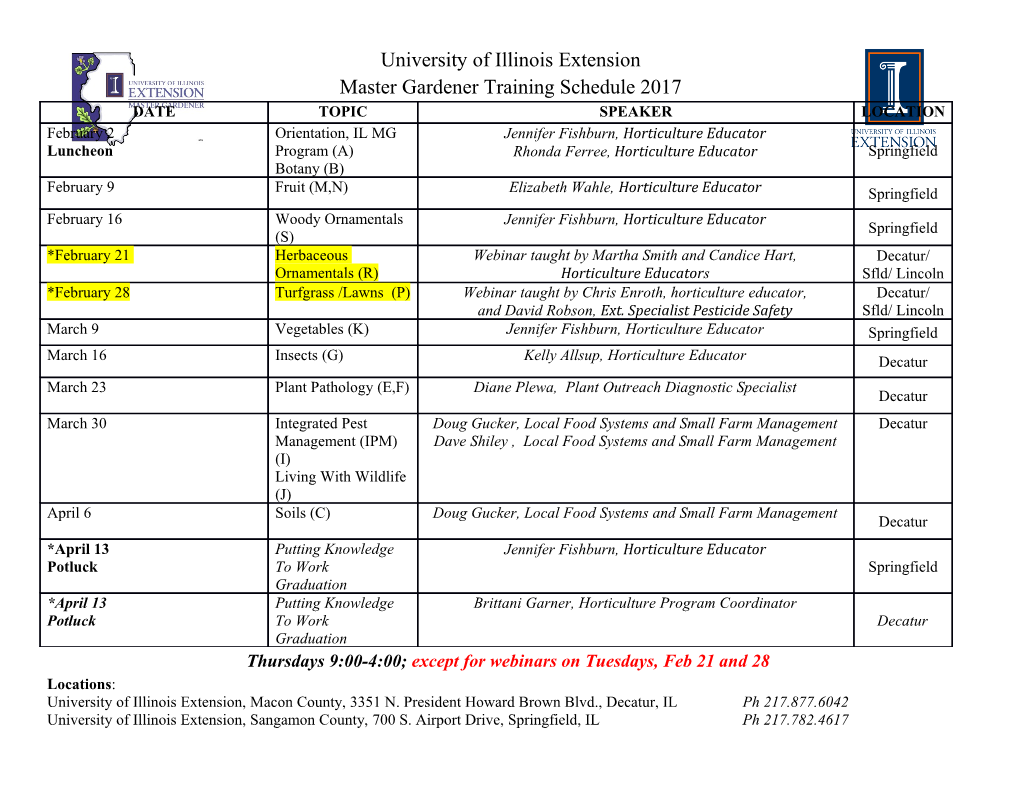
SEARCHING FOR A HEAVY PARTNER TO THE TOP QUARK JOSEPH VAN DER LIST 5e Abstract. We present a search for a heavy partner to the top quark with charge 3 , where e is the electron charge. We analyze data from Run 2 of the Large Hadron Collider at a center of mass energy of 13 TeV. This data has been previously investigated without tagging boosted top quark (top tagging) jets, with a data set corresponding to 2.2 fb−1. Here, we present the analysis at 2.3 fb−1 with top tagging. We observe no excesses above the standard model indicating detection of X5=3 , so we set lower limits on the mass of X5=3 . 1. Introduction 1.1. The Standard Model One of the greatest successes of 20th century physics was the classification of subatomic particles and forces into a framework now called the Standard Model of Particle Physics (or SM). Before the development of the SM, many particles had been discovered, but had not yet been codified into a complete framework. The Standard Model provided a unified theoretical framework which explained observed phenomena very well. Furthermore, it made many experimental predictions, such as the existence of the Higgs boson, and the confirmation of many of these has made the SM one of the most well-supported theories developed in the last century. Figure 1. A table showing the particles in the standard model of particle physics. [7] Broadly, the SM organizes subatomic particles into 3 major categories: quarks, leptons, and gauge bosons. Quarks are spin-½ particles which make up most of the mass of visible matter in the universe; nucleons (protons and neutrons) are composed of quarks. Quarks have color charge, and interact with each other via 1 the strong force. There are six quarks, three are up-type and three are down-type. The up-type quarks (up 2 (u), charm (c), and top (t)) have a charge of + 3 e, and the down-type quarks (down (d), strange (s), and 1 bottom (b)) have a charge of − 3 e. Composite particles which are made of quarks, like the proton, are called hadrons. Leptons are also spin-½ particles. Notably, leptons do not interact via the strong force as quarks do. One of the leptons, the electron (e), is one of the three components of the atom. The muon (µ) and the tau (τ) are particles which are similar to the electron, and share its charge of -e, but are heavier. The other three leptons are neutrinos. The electron, muon, and tau each have an associated neutrino, denoted νe, νµ, and ντ respectively. Neutrinos are very light neutral particles which rarely interact with other matter. They are primarily seen as products of reactions involving the weak nuclear force. The four gauge bosons are force-mediating particles with integer spins. The photon is massless and chargeless, and mediates the electromagnetic force. The gluon mediates the strong nuclear force while also carrying color charge, meaning that it also is affected by the strong force. The weak force is mediated by two different gauge bosons, the W and the Z. The W boson can have a charge of either +e or -e, while the Z boson is chargeless. The photon, W, and Z bosons can all also be considered the mediators of the eletroweak force, a unification of the electromagnetic and weak forces which occurs at high energies. The Higgs boson is the most recently discovered particle in the SM. From experiments, it is known that the bosons have mass, but in order for this to happen, theory requires that there exist a field with which the bosons can interact that breaks gauge symmetry. This field is called the Higgs field, and the Higgs boson is the excitation of this field. All of the particles in the SM have a corresponding particle of antimatter. These antiparticles have several important properties. Notably, the mass of any antiparticle is the same as the mass of the "regular" particle, while the electric and color charges are reversed. A particle and antiparticle can annihilate each other when they interact, and convert their mass to energy. Gluons, photons, and Z bosons are their own antiparticle, while W + and W − are antiparticles of each other. Antiparticles (when they are not the same as the regular particle) are denoted by a bar over the particle symbol. While the SM has great explanatory power, there are questions surrounding it which aren't yet answered. For example, there is no experimentally detected gauge boson which mediates the gravitational force. The search for physics which patch the holes in the SM is one of the most dynamic areas in high energy physics. 1.2. The X5=3 Particle In the summer of 2012, CMS and ATLAS announced the discovery of a new particle suspected to be, and now considered to be, the Higgs boson. The new particle, however, does not conform completely to the parameters expected from theory. Notably, it has a mass of about 125 GeV, while mass was expected to be much higher. The disparity between the expected and observed masses is an aspect of the hierarchy problem, one of the most fundamental problems yet to be solved surrounding the Higgs boson. In current predictions of the Higgs mass, known particles contribute terms which are divergent, so the calculated mass becomes very large. Within known physics, the only way to bring the predictions for the Higgs mass in line with observations is to invoke fine-tuning, i.e adjusting parameters of theory to very precise levels. Because fine-tuning is generally not considered to be satisfactory, new physics is sought to solve the problem. An appealing way to eliminate the problem of divergent terms from current particles is to invoke symmetry. The top quark, especially, contributes strongly to this effect and so symmetric partners to it are particularly interesting. One possible partner is a heavy quark with charge 5e/3 predicted by composite Higgs models, where the Higgs boson is not a fundamental particle but a composite one. This investigation deals with this heavy partner, which we call X5=3 . 1 Because we cannot directly detect the X5=3 particle , we must look for it indirectly. Theoretical predictions tell us about the decay of the X5=3 , so we can analyze recorded events in the detector to search for signatures which could be produced by an X5=3 . The presence of such signatures would indicate that there may have been an X5=3 created. 1 The X5=3 particle is very heavy and hence has a very short lifespan. Because of the short lifespan, an X5=3 will not have the chance to interact with the detector. 2 Figure 2. The pair production of an X5=3 and an X5=3 from a gluon. The newly created particles then decay in t + W + and t¯+ W − respectively. Figure 2 shows an example of the production and decay of X5=3 . Here, an X5=3 and an X5=3 are pair produced by a gluon and then decay into tW +tW¯ −. Afterwards, the top decays to bW + and the anti-top decays to ¯bW −.2 There are multiple channels which the process can follow after the top decays, and there are advantages and disadvantages to analyzing each one. For example, the hadronic channel, where all of the W bosons decay hadronically (e.g. W + ! cs¯) is the most likely, but intersects with a large amount of background which must be accounted for. On the other hand, the dileptonic channel, where two of the W's decay leptonically (into e, µ, or τ, plus a ν), is much less likely, but has a lower background. We investigate the "semi-leptonic" channel, where one of the W bosons decays leptonically while the rest decay hadronically. This channel is more likely than the dileptonic channel, but has less background than the hadronic channel, reaching a compromise between data volume and background presence. We further divide this channel into subchannels based on whether the lepton was an electron or muon. 1.3. Compact Muon Solenoid The Compact Muon Solenoid (CMS) is one of the two largest experiments being conducted at the Large Hadron Collider (LHC). The LHC is a 27 kilometer particle accelerator built near Geneva, Switzerland by CERN which is designed to accelerate protons close to the speed of light and collide them. Currently, the LHC is performing its second run, with a combined energy of its two proton bunches of 13 TeV in the center of mass of collisions. CMS is designed to measure events caused by these collisions in order to probe the physics of the high energy realm. The CMS detector is built in many layers, each of which performs a different function. Figure 3 shows a slice of the detector with the various layers. The innermost layer of the detector is composed of silicon trackers, essentially pieces of silicon between an anode and cathode. The trackers react to the passage of charged particles, giving information on their position. When a charged particle passes through a detector, it ionizes some of its atoms. The applied electric field then causes the electrons to drift to anode and cathode, giving a small current reading. The key feature of the trackers is that they are able to measure the position of particles passing through them very accurately. This means that the layer can be relatively light, and so interfere with the particles as little as possible. Ideally, the tracker layer measures the path of all charged particles through it without absorbing any energy from any of them.
Details
-
File Typepdf
-
Upload Time-
-
Content LanguagesEnglish
-
Upload UserAnonymous/Not logged-in
-
File Pages17 Page
-
File Size-