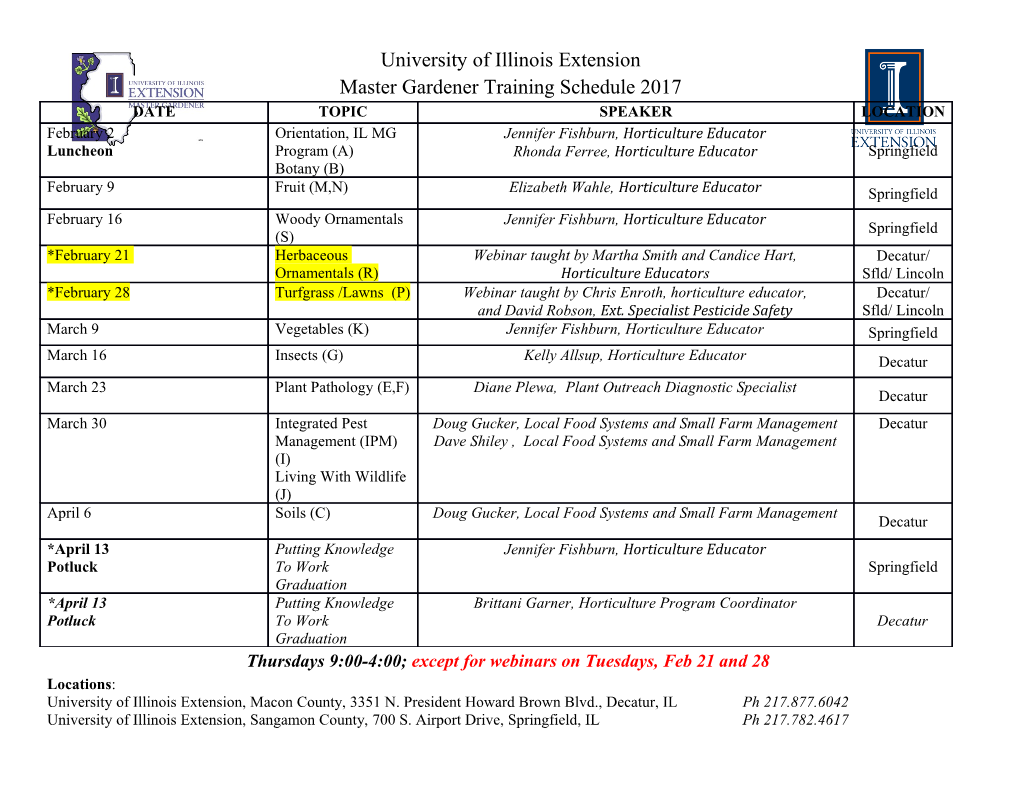
CHAPTER 18 Geochemical and Biological Consequences of Phytoplankton Evolution MIRIAM E. KATZ, KATJA FENNEL, AND PAUL G. FALKOWSKI I. Introduction A. The Two Carbon Cycles B. The “Great Oxidation Event” and the Wilson Cycle II. The Role of Phytoplankton in the Geological Carbon Cycle A. Early Phytoplankton Evolution B. The Rise of the Red Lineage C. Biological Overprint of the Geological Carbon Cycle III. The Phanerozoic Carbon Isotope Record δ13 A. Jurassic to Mid-Miocene 1.1% Ccarb Increase δ13 B. 2.5% Ccarb Decrease Since the Mid-Miocene IV. Feedbacks in Biogeochemical Cycles A. Phytoplankton Community Structure and the Wilson Cycle B. Biological Impact on Global Sedimentation Patterns C. Effects of Carbon Burial on Atmospheric Gases V. Concluding Remarks References I. INTRODUCTION Analyses of the stable isotopes of five of the six major light elements that comprise all The early evolution of Earth’s atmosphere, life on Earth (H, C, N, O, and S; there is only oceans, and lithosphere strongly influenced, one stable isotope of P) have provided clues and in turn was influenced by, the evolution about the co-evolution of life and geophysi- of life. Reconstructing the interactions and cal processes on Earth. Of these, the isotopic feedbacks between life and abiotic systems records of carbon and sulfur are perhaps the is one of the most challenging problems in most useful in reconstructing redox chem- understanding the history of the planet. istry resulting from biological evolution. 405 CCh18-P370518.inddh18-P370518.indd 440505 66/29/2007/29/2007 77:57:11:57:11 PPMM 406 18. GEOCHEMICAL AND BIOLOGICAL CONSEQUENCES OF PHYTOPLANKTON EVOLUTION Because these two elements are extremely up to four electrons and protons. Such abundant on the surface of the planet and reduction reactions are endergonic and do are capable of accepting and donating four not occur spontaneously on Earth’s surface and eight electron equivalents, respectively, without biological catalysis. The biologi- on geological time scales, their global bio- cally catalyzed reduction of CO2 invariably logical chemistry exerts a major control on leads to the production of an oxidant. If oxy- the redox conditions of the atmosphere and genic photosynthesis is the catalytic reac- oceans. In this chapter, we examine the role tion, then H2O is the source of the electrons of marine photoautotrophs on Earth’s car- and protons, and the oxidized water is con- bon cycle, with an emphasis on the impact verted to O2. In the steady state, the oxidant of these organisms on the redox changes produced by the photoautotrophs is rap- inferred from the isotopic signals preserved idly consumed (reduced) by heterotrophs in the geological record. to reoxidize the organic matter. However, if some small fraction of the organic matter is buried in sediments, it can escape biological A. The Two Carbon Cycles reoxidation; the buried organic matter is, in There are two major carbon cycles oper- effect, a sink of reductant. Electron balance ating on Earth. The “geological” (or “slow”) requires that if reductants are buried in the carbon cycle consists of a linked set of lithosphere, oxidants must be produced wholly abiotic, acid-base reactions in which somewhere else; in this case, this occurs in the atmosphere and oceans (Holland 1984). CO2 is outgassed primarily via volcanism, becomes hydrated to form carbonic acid, Hence, burial of organic matter implies oxi- and is subsequently consumed, primarily dation of the surface of Earth (Hayes et al. by reactions with magnesium- and calcium- 1999; Hayes and Waldbauer 2006). The biological reduction of CO to form bearing silicate rocks, to form magnesium 2 and calcium carbonates. The cycle is com- organic matter is invariably associated with pleted when the carbonates are subducted a large kinetic isotopic fractionation of up to along active continental margins, heated ~ 25‰. Hence, long-term burial of organic in the mantle, and outgassed again. In this matter depletes the atmosphere and ocean 12 13 cycle, no electron transfers occur and there of C, resulting in C-enriched carbonate are no significant changes in the isotopic pools (e.g., Broecker and Peng 1982; Kump compositions of the various carbon pools and Arthur 1999). Similarly, as long-term (Berner 2004). This geological carbon cycle, burial of organic matter depletes the atmos- 12 which is strictly dependent upon tectonic phere and ocean of C, organic matter 13 processes, operates on time scales of hun- becomes continuously enriched in C over dreds of millions of years; on such time time. These two fractionations are the basis scales, it is generally assumed that the bal- of the isotopic mass balance quantified as: ance between volcanism and weathering δ13 δ13 δ13 δ13 fw* Cw+fv* Cv= fcarb* Ccarb+forg* Corg (1) largely determines the CO2 concentration in Earth’s atmosphere (e.g., Berner, 1991). where f = fraction (i.e., a dimensionless ratio The “biological” (or “fast”) carbon cycle in which the sum of all “fs” in the equation is biologically driven and is based on redox equals 1.0), w = weathering processes, v = reactions, which are at the core the funda- volcanic/hydrothermal outgassing, carb = mental chemistry of life (Falkowski 2001). carbonate, and org = organic carbon. Redox reactions are characterized by trans- This equation is a biogeochemical fers of electrons with or without protons, analogue of the physical notion that, in the and like acid-base reactions, they always steady state, “what goes up, must come occur in pairs. CO2 is a potential electron down.” The left-hand side of the equa- sink; in its most reduced form, it can accept tion represents the input of carbon to the CCh18-P370518.inddh18-P370518.indd 440606 66/29/2007/29/2007 77:57:12:57:12 PPMM I. INTRODUCTION 407 atmospheric/oceanic/terrestrial ecosystems processes alter continental configuration from the lithosphere, and the right-hand and how that, in turn, influences the carbon side represents the output of organic mat- cycle and the oxidation state of Earth. ter and carbonates from the ocean and land It is generally believed there were to the lithosphere. Clearly, net oxidation of no continents in the early Archean Eon Earth’s atmosphere means that the equation (Drake and Righter 2002). The growth of cannot be in steady state; rather, the burial of continents required repeated subduction organic carbon must exceed its oxidation in of dense, basaltic mantle rocks, where this scenario (Berner and Canfield 1989). heating and recrystallization in the pres- The average isotopic value of mantle ence of water vapor produced silicate-rich CO2 is ca. −5‰, whereas that of organic (so-called felsic) rocks such as granites. matter is ca. −25‰. Hence, to balance the The granitic rock aggregated over time input with the output, the ratio of bur- into larger units to form cratons (the large, ied organic carbon relative to total carbon heterogenous lithopheric bases of conti- is about 0.20 to 0.25. That is, for every one nents), which are lighter than the under- atom of carbon buried as organic matter, lying mantle, and therefore float on that between four and five atoms are buried semifluid structure. as carbonate (see Guidry et al., Chapter 17, Cratons are thick, about three to four this volume). Hence, changes in marine times thicker than the denser basalts (called δ13 δ13 Ccarb and Corg through time serve as mafic rocks) that form the oceanic crusts. sedimentary archives of changes in carbon Near Earth’s surface, the heat that emanates sources and sinks, thereby providing the best from within the core is conducted about monitor available to reconstruct the geologi- three times more efficiently through the thin cal carbon cycle and its role in the oxidation oceanic crust than it is through the thicker state of the planet’s surface. continental crust. This differential heat dis- sipation leads to the build-up of thermal B. The “Great Oxidation Event” and the energy below large cratons, causing mas- Wilson Cycle sive pressures beneath them. As the heat and pressure build, the craton (continent) Although the evolution of oxygenic eventually thins and fractures, and pieces of photosynthesis provided a mechanism to continents slowly rift apart, creating a new produce large amounts of O2 from the ubiq- oceanic ridge spreading center between the uitous and abundant sources of reductant continental fragments. Heat-driven convec- (water) and energy (solar radiation), this tion in the mantle below the lithosphere process, in and of itself, was not sufficient to drives the tectonic plates apart with the frag- oxidize Earth’s atmosphere and oceans. Net mented continents attached, and new oce- oxidation requires net long-term burial of anic crust forms at the intervening spreading organic carbon in the lithosphere, where it center. The oceanic crust becomes cooler and is protected from reoxidation. Let us briefly denser as it ages and eventually subsides as examine how this process evolved. it moves away from the spreading center. The two basic physical processes involved When this old basaltic crust becomes so in continental evolution are the Archimedes dense that it subsides below the less dense Principle and heat conduction. The former adjacent continental crust, a new subduction holds that less dense bodies will float on zone is created, the whole process reverses more dense bodies; the latter suggests that itself, and the ocean basin is consumed as thicker, more insulated bodies do not con- the continents ultimately reassemble. The duct heat as efficiently as thinner, less insu- episodic break-up, dispersal, and subse- lated bodies. Let us put these two concepts quent reassembly of supercontinents occurs to work in understanding how tectonic over ~300–500 million year (myr) intervals CCh18-P370518.inddh18-P370518.indd 440707 66/29/2007/29/2007 77:57:12:57:12 PPMM 408 18.
Details
-
File Typepdf
-
Upload Time-
-
Content LanguagesEnglish
-
Upload UserAnonymous/Not logged-in
-
File Pages26 Page
-
File Size-