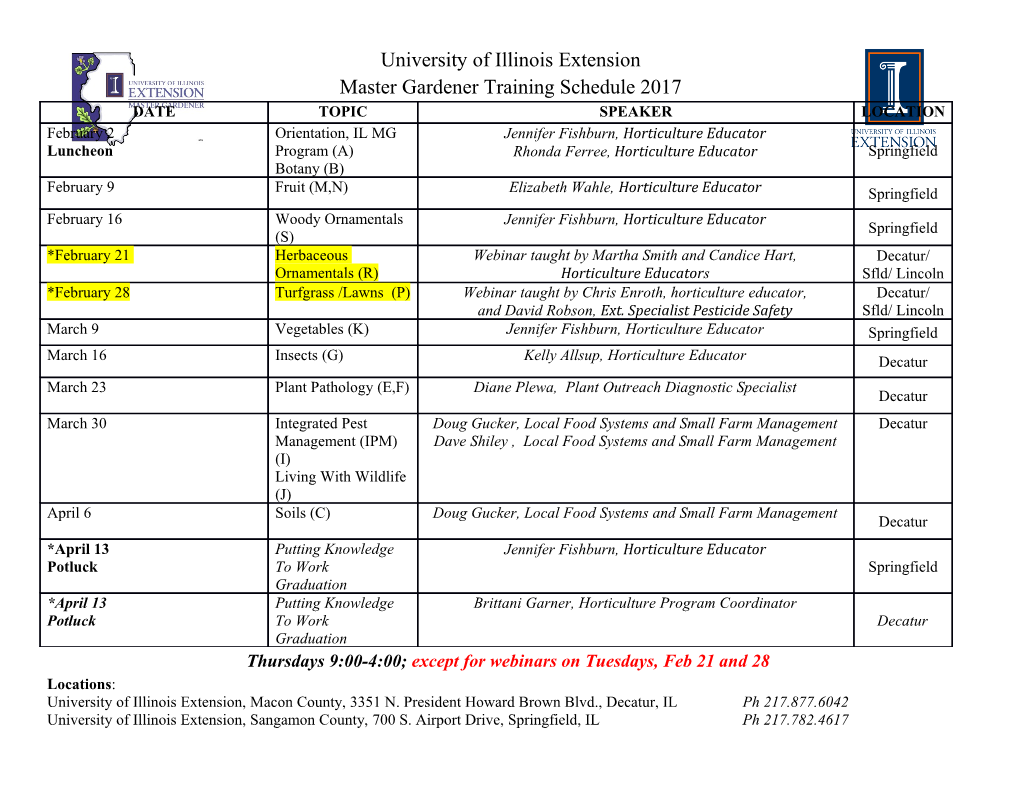
Poisson Structures and Integrability Peter J. Olver University of Minnesota http://www.math.umn.edu/ olver ∼ Hamiltonian Systems M — phase space; dim M = 2n Local coordinates: z = (p, q) = (p1, . , pn, q1, . , qn) Canonical Hamiltonian system: dz O I = J H J = − dt ∇ ! I O " Equivalently: dpi ∂H dqi ∂H = = dt − ∂qi dt ∂pi Lagrange Bracket (1808): n ∂pi ∂qi ∂qi ∂pi [ u , v ] = ∂u ∂v − ∂u ∂v i#= 1 (Canonical) Poisson Bracket (1809): n ∂u ∂v ∂u ∂v u , v = { } ∂pi ∂qi − ∂qi ∂pi i#= 1 Given functions u , . , u , the (2n) (2n) matrices with 1 2n × respective entries [ u , u ] u , u i, j = 1, . , 2n i j { i j } are mutually inverse. Canonical Poisson Bracket n ∂F ∂H ∂F ∂H F, H = F T J H = { } ∇ ∇ ∂pi ∂qi − ∂qi ∂pi i#= 1 = Poisson (1809) ⇒ Hamiltonian flow: dz = z, H = J H dt { } ∇ = Hamilton (1834) ⇒ First integral: dF F, H = 0 = 0 F (z(t)) = const. { } ⇐⇒ dt ⇐⇒ Poisson Brackets , : C∞(M, R) C∞(M, R) C∞(M, R) { · · } × −→ Bilinear: a F + b G, H = a F, H + b G, H { } { } { } F, a G + b H = a F, G + b F, H { } { } { } Skew Symmetric: F, H = H, F { } − { } Jacobi Identity: F, G, H + H, F, G + G, H, F = 0 { { } } { { } } { { } } Derivation: F, G H = F, G H + G F, H { } { } { } F, G, H C∞(M, R), a, b R. ∈ ∈ In coordinates z = (z1, . , zm), F, H = F T J(z) H { } ∇ ∇ where J(z)T = J(z) is a skew symmetric matrix. − The Jacobi identity imposes a system of quadratically nonlinear partial differential equations on its entries: ∂J jk ∂J ki ∂J ij J il + J jl + J kl = 0 ! ∂zl ∂zl ∂zl " #l Given a Poisson structure, the Hamiltonian flow corresponding to H C∞(M, R) is the system of ordinary differential equati∈ons dz = z, H = J(z) H dt { } ∇ Lie’s Theory of Function Groups Used for integration of partial differential equations: F , F = G (F , . , F ) { i j } ij 1 n = predates Lie groups!! ⇒ Ausgezeichnete Functionen = distinguished functions = Casimirs F, C = 0 for all F C∞(M, R) { } ∈ " " All distinguished functions are first integrals (conservation laws) of any associated Hamiltonian system. Darboux’ Theorem If J(z) has constant rank, then there exist local coordinates z = (p, q, y) such that the Poisson bracket is in canonical form n ∂F ∂H ∂F ∂H F, H = { } ∂pi ∂qi − ∂qi ∂pi i#= 1 Canonical degenerate Hamiltonian system: O I O dz = J H J = I −O O dt ∇ O O O Equivalently: dpi ∂H dqi ∂H dyj = = = 0 dt − ∂qi dt ∂pi dt Distinguished (Casimir) functions: F (y) = const. Variable rank: Weinstein, Conn. Symplectic Structures 2 A two form Ω V T M is called symplectic if it is ∈ ∗ closed: dΩ = 0, and • nondegenerate: Ω Ω = 0. • ∧ · · · ∧ + Nondegeneracy implies that Ω defines an isomorphism T M T M, mapping , ∗ the Hamiltonian vector field vH to dH = vH Ω. In coordinates Ω = dzT J(z)−1dz and v = J(z) H(z) ∂ . ∧ H ∇ z The associated nondegenerate Poisson bracket: F, H = v v ; Ω = F T J(z) H { } - F ∧ H . ∇ ∇ Lie–Poisson Structure Originally due to Lie • Rediscovered by Kirillov, Kostant, Souriau, . • g — r-dimensional Lie algebra M = g Rr — dual vector space ∗ , F, H = z ; [ F (z), H(z) ] { } - ∇ ∇ . z g F (z), H(z) C∞(g , R) ∈ ∗ ∈ ∗ F (z) g [ F (z), H(z) ] — Lie bracket in g ∇ ∈ ∇ ∇ Lie–Poisson Structure g — r-dimensional Lie algebra M = g Rr — dual vector space ∗ , Lie–Poisson bracket: F, H = z ; [ F (z), H(z) ] { } - ∇ ∇ . z g F (z), H(z) C∞(g , R) ∈ ∗ ∈ ∗ F (z) g [ F (z), H(z) ] — Lie bracket in g ∇ ∈ ∇ ∇ r In coordinates: J (z) = ck z , z = z µ1 + z µr g ij ij k 1 · · · r ∈ ∗ k#= 1 k cij — structure constants µ1, . , µr — Maurer–Cartan forms The Euler Equations = Arnold ⇒ Let G = SDiff(M) be the infinite-dimensional pseudo-group of volume preserving diffeomorphisms of a Riemannian manifold M. Lie algebra: g = divergence-free vector fields. 2 Hamiltonian functional on g∗: the L norm. " The corresponding Lie–Poisson flow is equivalent to the Euler equations governing the motion of an incompressible fluid. In Rn: u + u u = p div u = 0 t · ∇ − ∇ u — fluid velocity p — pressure = Ebin, Marsden ⇒ Vorticity Equations u — fluid velocity ω = u — vorticity ∇ ∧ ∂ω δH = ω u u ω = J ∂t · ∇ − · ∇ δω 1 2 H = 2 u dx — kinetic energy * | | J = ( ω ω ) — Poisson operator · ∇ − ∇ ∇ ∧ · Distinguished (Casimir) functions: 2D: f(ω) dx dy 3D: (u ω) dx dy dz — helicity * * * * * · Camassa–Holm Equation and Euler–Poincar´e Flows Use the H1 norm as the Hamiltonian for the Lie–Poisson struc- ture on the diffeomorphism pseudo-group: [u] = ( u2 + α u 2 ) dx H * | ∇ | Camassa–Holm Equation: u u = 3 u u (u u + 1u2) t ± xxt x ± xx 2 x x = nonanalytic solutions — peakons or compactons ⇒ α models: regularized Euler; geophysics, magnetohydrodynam- ics, computational anatomy,mathematical morphology Poisson Bivector Field = Lichnerowicz ⇒ T 2 Θ = ∂ J(z) ∂ V T M z ∧ z ∈ Poisson bracket: F, H = Θ ; dF dH { } - ∧ . " Bilinearity, skew symmetry and derivation properties are automatic. Theorem. The Jacobi identity holds if and only if [ Θ, Θ ] = 0 where [ , ] denotes the Schouten bracket. · · The Schouten Bracket The Schouten bracket [ Θ, Ψ ] is the natural extension of the Lie n bracket [ v, w ] to multi-vector fields (sections of ^ T M): Bilinear. • Super-symmetric: • deg Θ deg Ψ deg Θ+deg Ψ−1 [ Θ, Ψ ] = ( 1) [ Ψ, Θ ] ^ T M − ∈ Super-Jacobi identity: • ( 1)deg Θ deg Ξ [ [ Θ, Ψ ], Ξ ] + ( 1)deg Ξ deg Ψ [ [ Ξ, Θ ], Ψ ]+ − − + ( 1)deg Ψ deg Θ [ [ Ψ, Ξ ], Θ ] = 0 − Super-derivation: • [ Θ, Ψ Ξ ] = [ Θ, Ψ ] Ξ + ( 1)deg Ψ(deg Θ−1)Ψ [ Θ, Ξ ] ∧ ∧ − ∧ The Poisson Complex δΘ δΘ δΘ 0 - 1 - 2 - R ^ T M ^ T M ^ T M → · · · Suppose Θ is a Poisson bivector: [ Θ, Θ ] = 0 Poisson derivation: δΘ(Ψ) = [ Θ, Ψ ] Closure: By super-Jacobi: δ2 (Ψ) = [ Θ, [ Θ, Ψ ] ] = [ Θ, [ Θ, Ψ ] ] ( 1)deg Ψ[ Ψ, [ Θ, Θ ] ] = 0 Θ − − − In particular, applying δΘ to a function gives the associated Hamiltonian vector field: δΘ(H) = [ Θ, H ] = vH Poisson Cohomology Unless Θ is nondegenerate, the Poisson complex is not locally exact. If Θ has constant rank, the local cohomology involves the distinguished functions and forms. " Poisson cohomology is poorly understood in general. A nondegenerate Θ defines an algebra isomorphism k k ^ T M ^ T M via ω v = ω Θ. In this case, the , ∗ /−→ Poisson complex is isomorphic to the de Rham complex: d d d R 0 - 1 - 2 - ^ T ∗M ^ T ∗M ^ T ∗M → 6 6 6 · · · δΘ δΘ δΘ 0 - 1 - 2 - R ^ T M ^ T M ^ T M → · · · BiHamiltonian Systems Definition. A system of first order differential equations is called biHamiltonian if it can be written in Hamiltonian form in two distinct ways: du = J H = J H dt 1∇ 1 2∇ 0 Thus both J1 and J2 define Poisson brackets, which we assume are not constant multiples of each other. = Both Hamiltonians H and H are conserved. ⇒ 1 2 Infinite Toda Lattice 1 2 qi−1−qi H = ( 2 pi + e ) #i 1 (qi−1−qi)/2 1 a = e b = p − = Flaschka i 2 i 2 i 1 ⇒ dai dbi 2 2 = a (b b ) = 2(a a − ) dt i i+1 − i dt i − i 1 2 1 2 H1 = ( ai + 2 bi ) H0 = bi #i #i 1 0 a(T+ 1) 2a(T+ T−)a a(T+ 1)b J1 = − J2 = − 2 − 2 ! (1 T−)a 0 " ! b(1 T−)a 2(a T T−a ) " − − + − T+, T− — shift operators Compatibility J , J , and J + J are all Poisson • 1 2 1 2 The Poisson bivectors satisfy • [Θ1, Θ1] = [Θ2, Θ2] = [Θ1, Θ2] = 0 The recursion operator R = J J −1 satisfies the Nijenhuis torsion • 2 1 condition R2[ v, w ] R[ Rv, w ] R[ v, Rw ] + [ Rv, Rw ] = 0 − − The symplectic forms satisfy • −1 −1 −1 dΩ1 = dΩ2 = d(Ω1 + Ω2 ) = 0 Theorem. (Magri) Suppose du = J H = J H dt 1∇ 1 2∇ 0 is a biHamiltonian system, where J1, J2 form a compatible pair of Hamiltonian operators. Assume that J1 is nondegenerate, and define the recursion operator −1 R = J2J1 . Then there exist an infinite sequence of conserved Hamiltonians H0, H1, H2, . such that Each associated flow is a biHamiltonian system • du = F = J H = J H − = R F − dt n 1∇ n 2∇ n 1 n 1 The Hamiltonians are in involution with respect to either Poisson bracket: • H , H = 0 = H , H { n m }1 { n m }2 and hence conserved by all flows. The flows mutually commute. • Proof : Recursion: Starting at n = 1, the nth flow comes from the vector field vn = [ Θ2, Hn−1 ] = [ Θ1, Hn ] Set vn+1 = [ Θ2, Hn ]. Then, by super-Jacobi, compatibility, and closure [ Θ , v ] = [ Θ , [ Θ , H ] ] = [ Θ , [ Θ , H − ] ] [ [ Θ , Θ ], H − ] 1 n+1 1 2 n − 2 1 n 1 − 1 2 n 1 = [ Θ , v − ] = [ Θ , [ Θ , H − ] ] = 0 − 2 n 1 − 2 2 n 2 Then, by exactness of the Θ1–Poisson complex vn+1 = [ Θ1, Hn+1 ] for some Hn+1. Conservation: Using compatibility: v (H ) = [ v , H ] = [ [ Θ , H − ], H ] = [ H − , [ Θ , H ] ] n m n m 2 n 1 m − n 1 2 m = [ H − , [ Θ , H ] ] = [ [ Θ , H − ], H ] = v − (H ) − n 1 1 m+1 1 n 1 m+1 n 1 m+1 and repeat . to reduce to vn(Hn) = vn(Hn−1) = 0.
Details
-
File Typepdf
-
Upload Time-
-
Content LanguagesEnglish
-
Upload UserAnonymous/Not logged-in
-
File Pages65 Page
-
File Size-