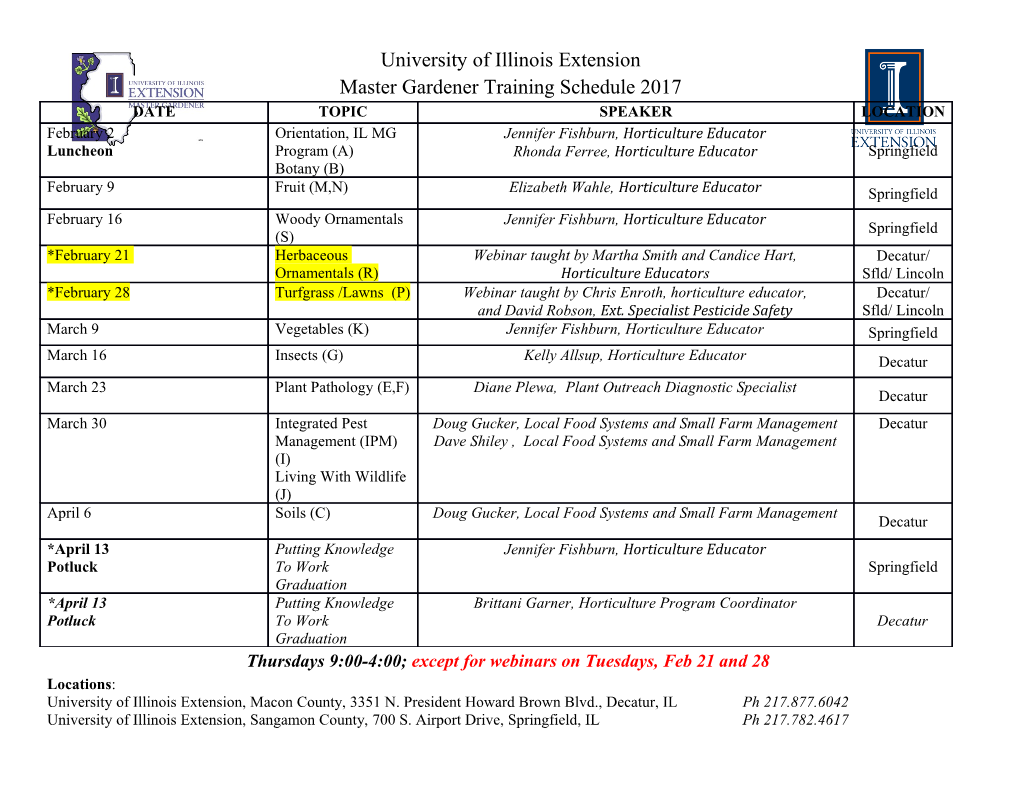
Clinical Applications of High-Energy Electrons 135 7 Clinical Applications of High-Energy Electrons Bruce J. Gerbi CONTENTS 7.8 Special Electron Techniques 159 7.8.1 Electron Arc Irradiation 159 7.1 Introduction 135 7.8.2 Craniospinal Irradiation 160 7.2 Historical Perspective 135 7.8.3 Total Skin Electron Therapy 162 7.3 Electron Interactions 136 References 164 7.4 Central Axis Percentage Depth-Dose Distributions 137 7.4.1 Central Axis Percentage Depth–Dose Dependence on Beam Energy 138 7.4.2 Central Axis Percentage Depth–Dose 7.1 Dependence on Field Size and SSD 138 7.4.3 Flatness and Symmetry – Introduction Off-Axis Characteristics 139 7.5 Isodose Curves 140 The basic physics of electron beams has been dis- 7.5.1 Change in Isodose Curves Versus SSD 141 cussed in several books and in several excellent 7.5.2 Change in Isodose Curves Versus Angle of chapters of standard radiation therapy textbooks Beam Incidence 142 Khan Hogstrom Strydom 7.5.3 Irregular Surfaces 142 ( 2003; 2004; et al. 2003). 7.6 Effect of Inhomogeneities on As with many of these previous works, the purpose Electron Distributions 143 of this chapter is to discuss the role of electron 7.6.1 Lungs 144 beams in radiation therapy, describe their physical 7.6.2 Bones 144 characteristics, and describe how this information is 7.6.3 Air Cavities 145 7.7 Clinical Applications of Electron Beams 146 relevant to clinical practice in radiation therapy. In 7.7.1 Target Definition 146 addition, several techniques using electron beams 7.7.2 Therapeutic Range – Selection of Beam Energy 147 that have been found to be useful in clinical settings 7.7.3 Dose Prescription – ICRU 71 147 will also be presented. 7.7.3.1 ICRU 71 Recommendations – Intraoperative Radiation Therapy 147 7.7.3.2 ICRU 71 Recommendations – Total Skin Irradiation 148 7.7.4 Field Shaping and Collimation 148 7.2 7.7.5 Internal Shielding 149 Historical Perspective 7.7.6 Bolus 151 7.7.6.1 Custom, Compensating Bolus 151 7.7.6.2 Field Abutment 152 In modern radiation therapy departments, high- 7.7.6.3 Electron–Electron Field Matching: energy electrons are a useful and expected modality. Sloping, Curved Surfaces 155 Although, they have been available for many years, 7.7.6.4 Electron–Photon Field Matching 156 it was not until the 1970s when linear accelerators 7.7.7 Intracavitary Irradiation 157 7.7.7.1 Intraoral and Transvaginal Irradiation 157 became widely available that electrons moved into 7.7.7.2 Intraoperative Radiation Therapy 158 the mainstream in radiation therapy. Similar to advances in photon beam treatments in radiation therapy, there have been several key advances in the 1970s that improved dramatically the ability to deliver optimized electron beam treatments. These developments were: (1) computed tomography (CT) B. J. Gerbi, PhD, Associate Professor Therapeutic Radiology – Radiation Oncology, University of scanners that paved the way for CT-based treat- Minnesota, Mayo Mail Code 494, 420 Delaware St SE, ment planning, (2) improvements in electron treat- Minneapolis, MN 55455, USA. [email protected] ment-planning algorithms (electron pencil beam 136 B. J. Gerbi algorithms) to calculate accurately and display dose rate of this decrease is about 2 MeV/cm in unit den- deposition using CT data, and (3) improvement in sity material such as muscle. linac designs resulting in improved depth dose, off- axis uniformity, and the physical characteristics of electron beams. Central to these latter improve- ments were the development of dual scattering foil 7.3 systems and improvements in electron beam appli- Electron Interactions cators (Hogstrom 2004). Modern linear accelerators are capable of pro- Two basic properties of electrons are that they pos- ducing several electron beam energies in addition sess a negative charge (we are not considering posi- to two or more photon energies. Electron beams trons at this time) and that they are low in mass in the range of 6–20 MeV are most clinically useful having approximately 1/2000 of the mass of a proton with the intermediate beam energies being the most or neutron. Being charged particles, they are directly commonly used. The energy designation of a clinical ionizing, meaning that they interact directly with beam is described using the most probable energy at the material on which they are incident. They are the surface of the phantom at the standard treatment attracted to charges of opposite sign and are repelled or source–skin distance (SSD). This most probable by charges of like sign as they travel through a energy is the energy possessed by the majority of the medium. These forces of attraction or repulsion are incident electrons and is represented on the linear called Coulomb force interactions and lead directly accelerator console by the closest integer value to the to ionizations and excitations of the absorbing mate- actual electron beam energy. Figure 7.1 shows the rial. Due to their relatively low mass, their direction nature of the electron energy spectrum as a func- of travel can be changed easily during these interac- tion of location in the beam. Before the accelerator tions. When electrons pass through a medium, their beam exit window, the electrons are fairly monoen- mean energy decreases with depth and they scatter ergetic in nature. The spectrum of electron energies to the side of their original path. Thus, the locations in the beam is spread more widely as it hits the sur- to which electrons scatter dictates where bonds are face of the phantom with the spectrum centered on broken and ultimately where dose is deposited. the most probable energy. As the beam penetrates Interactions undergone by electrons can be either into the patient, the width of the energy spectrum elastic in which no kinetic energy is lost or inelas- increases while the average energy of the beam tic in which some portion of the kinetic energy is decreases with increasing depth in the medium. The changed into another form of energy. Elastic colli- Phantom Filter Window Accelerator z at depth z E0 at surface before Z = 0 window Fig. 7.1. Representation of the energy of electrons in a linear accelerator beam as a function of the location in the beam. Ep,o represents the most probable energy at a – / arbitrary units E depth of zero, the surface; E0 is the aver- ) age mean energy at the surface; and Ep,z is (Emax)0 the most probable energy at the depth, z. [Reprinted with permission from Brahme and Svensson (1976). Specifi cation of elec- (Ep)z (Ep)0 Ea tron beam quality from the central-axis E / arbitrary units depth absorbed-dose distribution.] Clinical Applications of High-Energy Electrons 137 sions occur with either atomic electrons or atomic Rq nuclei, resulting in elastic scattering. These inter- Dm actions are characterized by a change in direction 90 R of the incident electron with no loss of energy. In Ds p G0 = the collision process between the incident electron Rp – Rq and atomic electrons, it is possible for the ejected electron to acquire enough kinetic energy to cause 50 additional ionizations of its own. These electrons are called secondary electrons or delta rays and they can go on to produce additional ionizations Absorbed Dose (%) and excitations. Inelastic collisions can occur with electrons resulting in ionizations and excitations of atoms or inelastic collisions of nuclei that result in Dx the production of Bremsstrahlung X-rays (radiative 12 4 56 R R R losses). The energy of these X-rays can be as high 0.05 100 Rt 50 p as the maximum accelerating potential of the beam Depth in Water (cm) and contribute dose to tissues deep within the body. Fig. 7.2. Central-axis depth–dose curve for an electron beam The magnitude of this photon contamination com- with parameters indicated that can be used to characterize ponent can range from about 1% to as high as 5% for electron beams. The therapeutic range is at the 90% depth– 6-MeV and 20-MeV electron beams, respectively. dose level and all other parameters are discussed in ICRU (1984) The typical energy loss in tissue for a therapeu- tic electron beam, averaged over its entire range, is about 2 MeV/cm in water. The rate of energy loss for while Dx is the dose due to X-ray contamination. collisional interactions depends on the energy of the R100 is the depth of the maximum dose while R90 is electrons and on the electron density of the medium. the depth of the 90% depth dose. R50 is the depth 2 The rate of energy loss per gram/cm is greater for of the 50% depth–dose curve. Rp is the practical low atomic number (low Z) materials than for high range, which is close to the most probable energy Z materials. This is because high Z materials have of the electron beam at the surface, E0, divided by fewer electrons per gram than low Z materials. In two in centimeters. Thus, a 20-MeV electron beam addition, the electrons in high Z materials are more would have a practical range of 10 cm in tissue. Rp tightly bound and are thus not available for these is determined by taking the depth of intersection types of interactions. Keeping the above types of of the straight line descending portion of the cen- interactions of electrons in mind will help to explain tral axis percentage depth–dose curve with a line many of the clinical situations presented later. The drawn representing the photon contamination.
Details
-
File Typepdf
-
Upload Time-
-
Content LanguagesEnglish
-
Upload UserAnonymous/Not logged-in
-
File Pages31 Page
-
File Size-