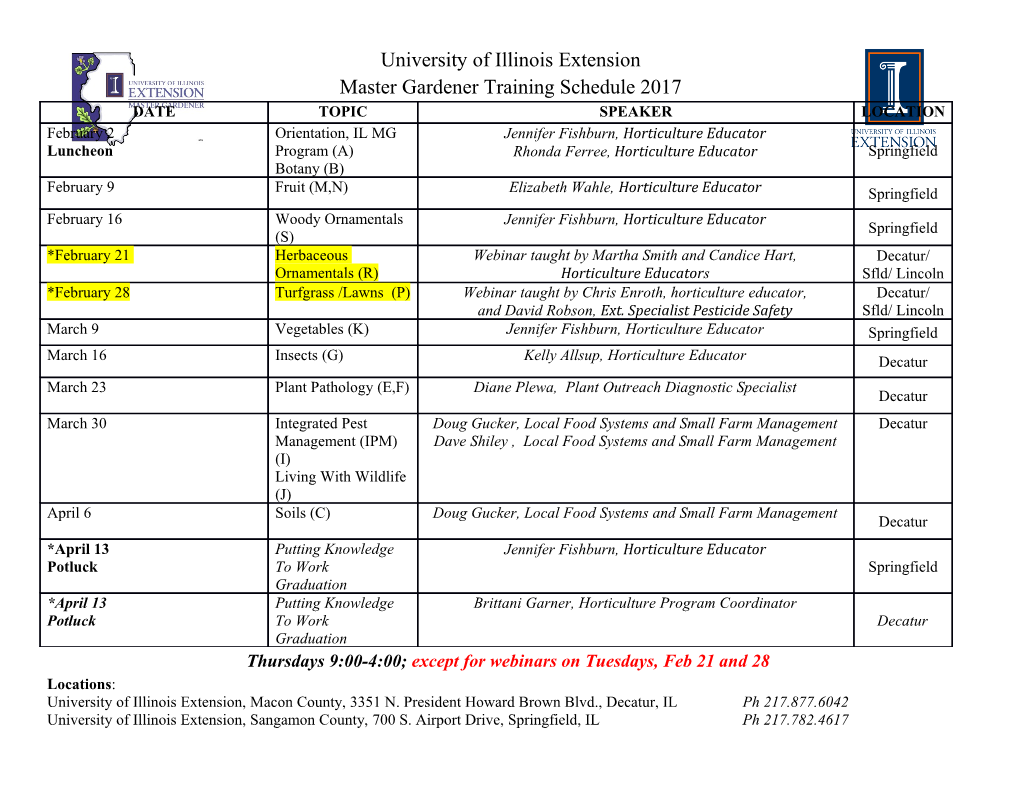
bioRxiv preprint doi: https://doi.org/10.1101/2021.01.05.425035; this version posted January 5, 2021. The copyright holder for this preprint (which was not certified by peer review) is the author/funder, who has granted bioRxiv a license to display the preprint in perpetuity. It is made available under aCC-BY-NC 4.0 International license. Cell migration driven by long-lived spatial memory Joseph d'Alessandro∗yz, Alex Barbier-Chebbahxy, Victor Cellerin,∗ Olivier B´enichou ,x Ren´e-MarcM`ege,∗ Rapha¨elVoituriez{z, and Beno^ıtLadoux∗z 1 Many living cells actively migrate in their environment to perform key biolog- 2 ical functions { from unicellular organisms looking for food to single cells such 3 as fibroblasts, leukocytes or cancer cells that can shape, patrol or invade tis- 4 sues. Cell migration results from complex intracellular processes that enable 1,2 5 cell self-propulsion , and has been shown to also integrate various chemi- 3,4,5 6 cal or physical extracellular signals . While it is established that cells can 7 modify their environment by depositing biochemical signals or mechanically 8 remodeling the extracellular matrix, the impact of such self-induced environ- 9 mental perturbations on cell trajectories at various scales remains unexplored. 10 Here, we show that cells remember their path : by confining cells on 1D and 11 2D micropatterned surfaces, we demonstrate that motile cells leave long-lived 12 physicochemical footprints along their way, which determine their future path. 13 On this basis, we argue that cell trajectories belong to the general class of self- 14 interacting random walks, and show that self-interactions can rule large scale 15 exploration by inducing long-lived ageing, subdiffusion and anomalous first- 16 passage statistics. Altogether, our joint experimental and theoretical approach 17 points to a generic coupling between motile cells and their environment, which 18 endows cells with a spatial memory of their path and can dramatically change 19 their space exploration. 20 Cell migration is essential for fundamental phases of development and adult life, including 7 21 embryogenesis, wound healing, and inflammatory responses ; it generically results from 22 the active dynamics of its intracellular components { most prominently the cytoskeleton 8,9 23 {, which generate propulsion forces and determine the cell front-rear polarity . The 10 24 cytoskeleton spatio-temporal dynamics is controlled by complex regulatory networks , ∗Institut Jacques Monod (IJM), CNRS UMR 7592 et Universit´ede Paris, 75013 Paris, France. yJdA and ABC contributed equally to this work zTo whom correspondence should be addressed: joseph.d'[email protected], voiturie@lptmc. jussieu.fr, [email protected]. xLaboratoire de Physique Th´eoriquede la Mati`ereCondens´ee,CNRS / Sorbonne Universit´e,4 Place Jussieu, Paris, France {Laboratoire Jean Perrin and Laboratoire de Physique Th´eoriquede la Mati`ereCondens´ee,CNRS / Sorbonne Universit´e, 4 Place Jussieu, Paris, France 1 bioRxiv preprint doi: https://doi.org/10.1101/2021.01.05.425035; this version posted January 5, 2021. The copyright holder for this preprint (which was not certified by peer review) is the author/funder, who has granted bioRxiv a license to display the preprint in perpetuity. It is made available under aCC-BY-NC 4.0 International license. 11,12,13,14 25 and can be characterized by both deterministic and stochastic components . The 26 integration over time of this complex intracellular dynamics determines the large scale 27 properties of cell trajectories, which can in turn be used as accessible read-outs to infer 2,15 5,16 28 intracellular properties , as well as cell interactions with the environment or with 17,18,19 29 neighbouring cells . In vivo, cells interact with various extra-cellular environments 8 30 with a broad range of biochemical and biomechanical properties . These interactions have 31 been shown to be two-way: environmental cues directly affect cell shape, migration, and 20,21,22 6,23 32 polarity , and in turn, cells actively contribute to remodel their environment . 33 So far, however, both ways have been analysed independently, and the feed-back of cell- 34 induced environmental remodeling on large scale properties of cell migration has remained 35 unexplored. 36 To overcome the inherent complexity of the analysis of cell migration in 3D in vivo environ- 24,25,26 37 ments, the design of micropatterned surfaces has proven to be a powerful approach . 38 In such in vitro set-ups, and especially in 1D settings, the reduced dimensionality of the cel- 39 lular environment allows for an extensive quantitative analysis of the phase space roamed 40 by migrating cells. In particular, such 1D assays have revealed striking deterministic fea- 41 tures in cell motility patterns, while cell paths in higher dimensions remain seemingly 27,14,28,6,19 42 random . Moreover, many of the cell migration features on a 1D substrate can 6 43 mimic cell behaviour in 3D matrix . 44 To dissect the mechanisms driving the spontaneous migration of living cells over a broad 45 range of time scales, we followed single isolated MDCK epithelial cells (treated with mito- 46 mycin C to prevent cell division) on micro-contact-printed 1D linear tracks of fibronectin 47 (Figure 1a-b). This set-up allowed us to track cells from their early spreading phase and 48 on over long time scales (48 − 96 h) using video-microscopy (Figure 1b-c). By detecting 49 the cell edges, we could reconstruct the trajectories of single cells in absence of cell-cell 50 contact interactions. We observed two main behaviours: a first population of cells exhib- 51 ited static spreading, and extended slowly their two ends in opposite directions without 52 net displacement of their centre-of-mass (Figure 1d and Supplementary Video 1), while a 53 second population displayed strikingly regular oscillatory trajectories, with an amplitude 54 that could significantly exceed the cell size (Figure 1e and Supplementary Video 2). While 29,30 31,32,33 55 oscillatory patterns in cell migration , and more generally in cell dynamics , have 56 been reported for various cell types, and could be attributed to different intracellular pro- 57 cesses, we argue below that the oscillations that we observe have so far unrevealed features, 58 which we show originate from a so far unreported mechanism. Both observed behaviours 59 were approximately equally distributed over the cell population, whereas behaviours that 60 did not fall in these two classes { akin to persistent random motion { remained negligible. 61 These observations were robust upon varying the width of the track over a range compa- 2 bioRxiv preprint doi: https://doi.org/10.1101/2021.01.05.425035; this version posted January 5, 2021. The copyright holder for this preprint (which was not certified by peer review) is the author/funder, who has granted bioRxiv a license to display the preprint in perpetuity. It is made available under aCC-BY-NC 4.0 International license. 62 rable to a single cell size, W = 10; 20; 50 µm (Figure 1f and Supplementary Figure 1a{c). 63 To analyse the dynamics of front back cell polarity in these motility patterns, we used 64 as a proxy for cell polarisation the concentration profile of a fluorescent biosensor (p21- 24 65 activated kinase binding domain, PBD) of active Rac1 and Cdc42 , which are two well 66 known activators of actin protrusions ; as expected, moving cells displayed an increased 67 PBD signal at the front, and lowered at the back, indicating their polarity. Two distinct 68 phenotypes of polarisation, corresponding to the two observed dynamic behaviours clearly 69 emerged from observations. On the one hand, static spreading cells were usually extended 70 (up to > 100 µm) and characterized by a symmetric PBD profile with active poles at 71 each of the two cell ends (see Supplementary Figure 1g and Supplementary Video 3). 72 On the other hand, oscillating cells displayed "run" phases characterized by reduced cell 73 length (∼ 20 µm), clear front back polarity, and roughly constant high speed (often faster −1 74 than 100 µm:h , see Figure 1f-g, Supplementary Figure 1 and Supplementary Video 3-4). 75 These run phases were interrupted by phases of polarity reversal, where the cell phenotype 76 was transiently comparable to that of static spreading cells. 77 We next focused on the oscillatory motility patterns, and characterized quantitatively 78 their striking regularity. Despite some heterogeneity within the population, pointing to 79 single-cell-specific properties, kymographs consistently displayed sawtooth-like patterns 80 (Supplementary Figure 1). Both the period and amplitude increased in time, starting 81 from very short (A ' 20 µm;T < 1 h) scales and reaching very high values up to A = 82 500 µm;T = 20 h (Supplementary Figure 2). Strikingly, within single trajectories the A=T 83 ratio remained close to constant over this very broad range of values of A and T . This 84 was consistent with cells running at roughly constant speed and bouncing between two 85 virtual reflecting walls imposing polarity reversals, which would slowly move apart. Based 86 on this observation, and because in our set-up external cues imposing such dynamics 87 could be excluded, we hypothesized that the observed polarity reversals were induced by 88 interactions of cells with their own footprints, and not caused only by an autonomous 89 intracellular clock. More precisely, we conjectured that cells modify the physicochemical 90 properties of their local environment, thereby leaving long-lived footprints along their path. 91 In turn, footprints were assumed to induce local polarity signals that favor cell polarisation 92 pointing towards previously visited areas, and away from unvisited areas, and thus to 93 effectively attract cells, thereby slowing down the large scale spreading of trajectories.
Details
-
File Typepdf
-
Upload Time-
-
Content LanguagesEnglish
-
Upload UserAnonymous/Not logged-in
-
File Pages18 Page
-
File Size-