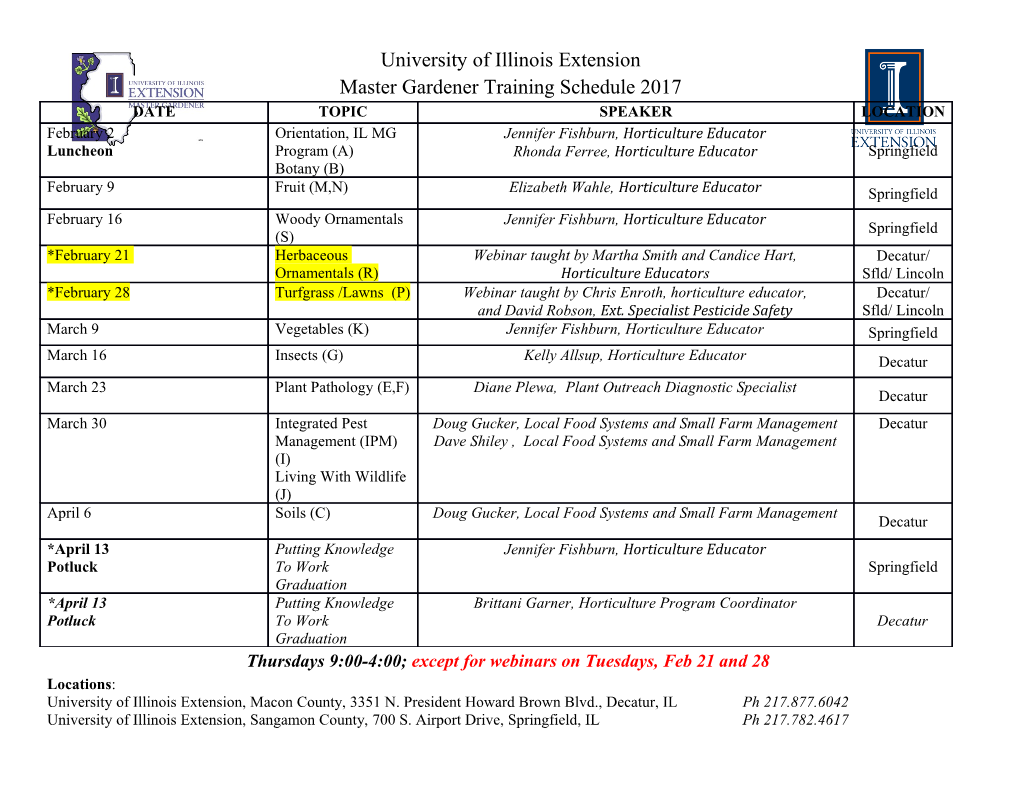
Scanning Microscopy Volume 1993 Number 7 Physics of Generation and Detection Article 16 of Signals Used for Microcharacterization 1993 Applications of Charge Collection Microscopy: Electron-Beam- Induced Current to Semiconductor Materials and Device Research Richard J. Matson National Renewable Energy Laboratory, Colorado Follow this and additional works at: https://digitalcommons.usu.edu/microscopy Part of the Biology Commons Recommended Citation Matson, Richard J. (1993) "Applications of Charge Collection Microscopy: Electron-Beam-Induced Current to Semiconductor Materials and Device Research," Scanning Microscopy: Vol. 1993 : No. 7 , Article 16. Available at: https://digitalcommons.usu.edu/microscopy/vol1993/iss7/16 This Article is brought to you for free and open access by the Western Dairy Center at DigitalCommons@USU. It has been accepted for inclusion in Scanning Microscopy by an authorized administrator of DigitalCommons@USU. For more information, please contact [email protected]. Scanning Microscopy Supplement 7, 1993 (Pages 243-251) 0892-953X/93$5.00+ .25 Scanning Microscopy International, Chicago (AMF O'Hare), IL 60666 USA APPLICATIONS OF CHARGE COLLECTION MICROSCOPY: ELECTRON-BEAM-INDUCED CURRENT TO SEMICONDUCTOR MATERIALS AND DEVICE RESEARCH Richard J. Matson National Renewable Energy Laboratory 1617 Cole Blvd., Golden, CO 80401 Phone No.: (303) 275-3726, FAX No.: (303) 275-3701 Abstract Introduction Among all of the possible techniques for the micro­ The many ways in which a high energy electron in­ characterization of semiconductor materials and devices teracts with matter give rise to a number of useful, ob­ in a scanning electron microscope (SEM), charge collec­ servable signatures of a sample in the scanning electron tion microscopy (CCM), more commonly known as microscope (SEM). Semiconducting materials and de­ electron-beam-induced current (EBIC), is probably both vices, in particular, lend themselves to a wide variety of the most easily deployed and the most versatile charac­ SEM-based analytical approaches. In particular: charge terization technique. Following a brief review of the collection microscopy (CCM), voltage contrast (VC), basic theory of the generation and detection of the EBIC cathodoluminescence (CL), electron channeling (EC), signals, various techniques and applications to the micro­ scanning deep-level transient spectroscopy (SDLTS), and characterization of experimental semiconductor materials scanning electron-acoustic (thermal wave) microscopy and devices will be presented. The applications are pri­ (SEAM). All of these have been reviewed in the book marily to emerging photovoltaic materials and devices. SEM Micro-Characterization of Semiconductors, edited This report is not in any way intended as a complete by D.B. Holt and D.C. Joy (1989). Of all of these overview of all EBIC related techniques or most material microcharacterization techniques, CCM may be the most or device applications. Beyond offering a short review easily deployed and the most powerful analytical tool for of CCM theory, this paper complements the literature characterizing electronic materials and devices. As a (1) as a basic introduction to CCM, and (2) by focusing practical introduction, it is the purpose of the present on the use and extension of CCM techniques for basic paper to briefly review the theory of the generation and studies in experimental electronic materials. detection of the CCM/EBIC (electron-beam-induced current) signal and then present a variety of recent extensions and applications of EBIC techniques. The terms EBIC and CCM will be used interchangeably in this paper because EBIC is the most commonly used term for this technique in the literature and in the community, although "EBIC" can be confused with elec­ tron-beam-induced conductivity and is thereby less pre­ cise than CCM (see Holt, 1974). Charge collection has Key Words: Charge collection IT1Jcroscopy (CCM), also been called the barrier electron voltaic effect and is electron-beam-induced current (EBIC), m1cro­ the least commonly used term in the literature characterization, photovoltaic, introduction. (Ehrenberg et al., 1981; Leamy, 1982; Holt, 1974; Holt, 1989). Generation and Detection of EBIC Signal When a semiconductor device is penetrated by ener­ getic ( > 1 ke V) primary electrons, the succession of scattering events, or "collisions," result in the promotion of electrons from the valence band to the conduction band, thereby producing electron-hole (e/h) pairs. Without the presence of an electric field, the electrons and holes simply recombine. If, as in the case of a 243 Richard J. Matson Scan direction a SEM ,.._________.A >------f B CRT b Figure l. A schematic illustration of the scanning movement of an electron beam across a polycrystalline device. The light lines indicate grain boundaries which usually act as recombination sites and, therefore, locations of current loss. semiconductor device, there is an electric field present within collection distance of the e/h creation, the elec­ tron and hole can be separated and collectec.l via contacts to the device. In tum, this collected current is amplified and used to modulate the intensity of the SEM CRT Figure 2. A comparison of an EBIC image (a) and the (cathode ray tube), as indicated in Figure 1. As an secondary electron image (SET) (b) of the same area of example, an EBIC image (a) and a secondary electron a MTS device on Wacker polycrystalline silicon. The image (SEI) (b) of a simple metal-insulator-semi­ areas of recombination (defects) show up dark in EBIC conductor (MTS) device made from polycrystalline images. Bar = 100 µm. silicon is given in Figure 2. The effect of grain boundaries, or other sources of recombination, on the charge collection image is evident. Wherever there is relative ease of creating such a Schottky barrier (often electron/hole recombination, there is a corresponding with the deposition of - 200 A of Au) that readily loss of collected current and, therefore, CRT intensity, extends the use of CCM to the investigation of which appears as the dark areas in the image. Note the developing electronic materials that are not yet devices. difference of the density of defects among different Considering e-/h + pair generation more closely, we grains and differing portions of grain boundaries. can calculate .0.N, the number of e/h pairs generated per Essentially, the electron beam acts as a constant, second for a given SEM beam condition, by mobile point source of current generation and can be used for "micro" -characterization, or characterization of (1) micron scale variations in the electrical properties of a material or device. where (2) Effectively, the sample itself is its own detector, and nothing other than amplification of the EBIC signal and and f is the average fraction of the energy of the incident a SEM vacuum chamber feedthrough for the signal is beam electrons lost to back-scattering [often taken as necessary to deploy this capability. Lacking an actual p­ half the backscatter coefficient (Holt, 1989)]; lb and Eb n junction in the device, the electric field required for are the electron beam current and voltage, respectively; charge separation can be provided through either an ex­ q is the electronic charge; ei is the ionization charge or ternal bias applied to the device or through the simple the average energy required to create an e/h pair; and G fabrication of a Schottky barrier device. It is the is the gain. A good value for ei is usually taken as 244 EBIC applications in semiconductor R & D approximately three times the Eg of the material (Holt, 1989; Leamy, 1982). By dividing the energy available in a primary electron for ionization by the energy re­ 1.0 quired for ionization, the gain, G, gives the factor by which the maximum EBIC can exceed the Jb, which 4 commonly runs in the range of lo'.3-10 . The practical significance of this is (a) that common Tb values of 100 pA can give rise to µA-range signals which can be han­ dled "cleanly" by most amplifiers, and (b) that even very inefficient experimental devices can still be charac­ terized quite often by CCM. In this author's experience, the lowest "clean," or useful, scale for amplifiers is the nA scale. Hence, even a 1 % efficient device and a 150-pA, 20-kV beam still leaves an adequate signal. Figure 3. EBIC line scan superimposed on an EBIC One use of the relation in equation (1) is to establish map of the defects in an epilayer of GaAs on a silicon a reference for the efficiency of the device. Termed substrate. The top line is the 100 % electron beam quan­ electron-beam quantum yield, or charge-collection effi­ tum efficiency reference line per equation 1. The bot­ ciency of a barrier, 11cc(Holt, 1989), the measured tom line is both the position of the line scan and the zero EBIC, ICC' is normalized to the theoretically generated beam current reference line. The middle line is the current, or EBIC linescan indicating the actual current loss in a defect area relative to 100% collection. Bar = 10 µm. (3) This is demonstrated in Figure 3 on an epilayer of a beam in Si, the injection levels are 1023 pairs/cm 3 /sec. GaAs-on-Si device where the atomic lattice mismatch re­ In addition, ESD processes scale directly with the charge sulted in dislocation networks. In Figure 3, the refer­ density (ibid.). In an earlier work, for example, the ence for 100 % quantum efficiency (per equation 1) is case was made that even with a 100-A, 20-kV, 50-pA represented by the top, horizontal line. The bottom line beam, the electron beam was desorbing oxygen from is both the position of the line scan and the zero beam polycrystalline CuTnSei, thereby locally type-converting current reference line. The middle line is the EBIC it from p-type ton-type (Matson et al., I 989).
Details
-
File Typepdf
-
Upload Time-
-
Content LanguagesEnglish
-
Upload UserAnonymous/Not logged-in
-
File Pages10 Page
-
File Size-