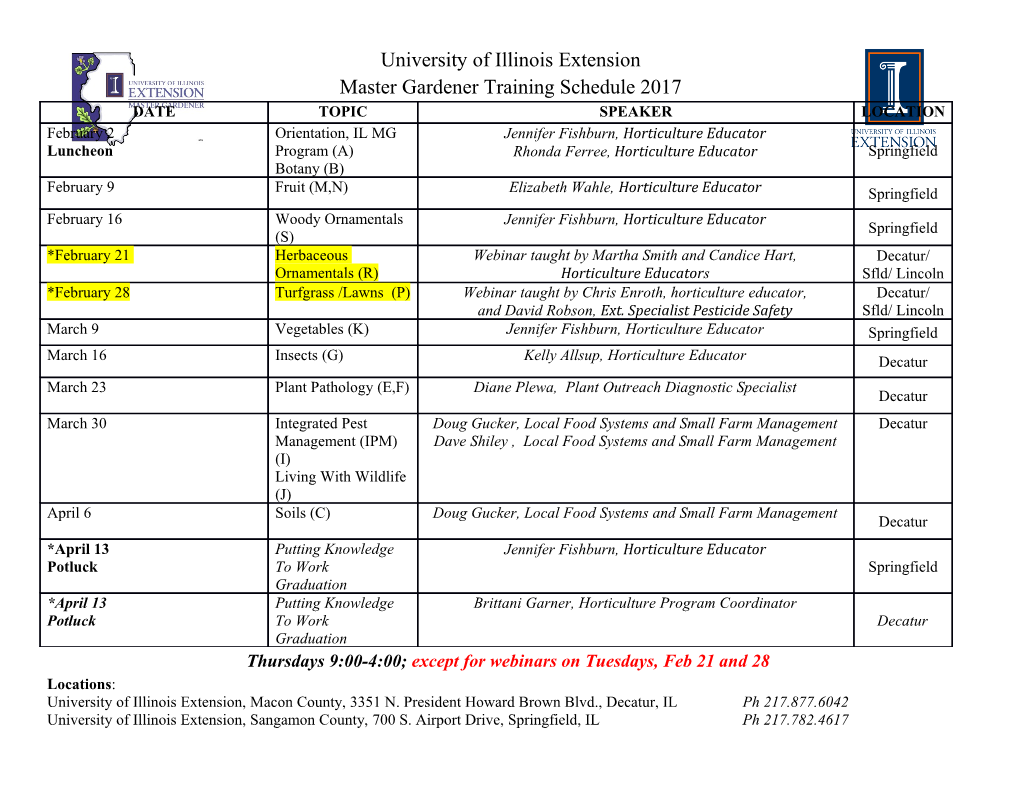
Deformation mechanisms in free-standing nanoscale thin films: A quantitative in situ transmission electron microscope study M. A. Haque* and M. T. A. Saif†‡ *Department of Mechanical and Nuclear Engineering, Pennsylvania State University, 317A Leonhard Building, University Park, PA 16802; and †Department of Mechanical and Industrial Engineering, University of Illinois at Urbana–Champaign, 140 MEB, 1206 West Green Street, Urbana, IL 61801 Edited by Jan D. Achenbach, Northwestern University, Evanston, IL, and approved March 1, 2004 (received for review January 5, 2004) We have added force and displacement measurement capabilities the TEM has so far been a major analytical instrument in in the transmission electron microscope (TEM) for in situ quanti- materials research (29), nonuniformity in specimen cross-section tative tensile experimentation on nanoscale specimens. Employing and space restrictions for the accommodation of force and the technique, we measured the stress–strain response of several displacement sensors have limited its application to qualitative nanoscale free-standing aluminum and gold films subjected to in situ straining without simultaneous measurement of stress– several loading and unloading cycles. We observed low elastic strain response (30–32). Our experimental setup consists of a modulus, nonlinear elasticity, lack of work hardening, and macro- free-standing tensile sample with uniform width and thickness, scopically brittle nature in these metals when their average grain cofabricated with a microtensile stage with force and displace- size is 50 nm or less. Direct in situ TEM observation of the absence ment sensors. They are made by microelectromechanical systems of dislocations in these films even at high stresses points to a (MEMS) fabrication technology based on lithography. Cofabri- grain-boundary-based mechanism as a dominant contributing fac- cation and the specific flexure design of the stage ensure tor in nanoscale metal deformation. When grain size is larger, the alignment between the loading and the specimen directions, and same metals regain their macroscopic behavior. Addition of quan- minimize the setup size. titative capability makes the TEM a versatile tool for new funda- Fig. 1 shows the microtensile test stage with a 100-nm-thick ENGINEERING mental investigations on materials and structures at the nano- aluminum specimen bonded to a force sensor beam at one end, scale. and to a set of supporting beams at the other end. The beams are made of single-crystal silicon (27). Quasistatic displacements are anomechanical behavior of materials has gained consider- imposed on one end of the stage and are then transmitted to the Nable attention because of the ever-shrinking dimensions of force sensor beam through the free-standing specimen. The thin-film materials in microelectronics, data storage, and micro- displacement sensors (in the form of two marker gaps, F and D) electromechanical sensors͞actuators, and also the advancements read the specimen elongation. The force on the specimen is given in bulk nanostructured materials. Mechanical properties of by the spring constant and the displacement (marker F) of the materials are influenced by their length-scales. For example, force sensor beam. The spring constant is calibrated with a yield stress of polycrystalline metals increases with a decrease in nanoindenter after the tensile experiment is completed. The grain size [Hall–Petch behavior (1, 2)], and strain-gradient- supporting flexural beam assembly prevents misalignment of the dependent strengthening (3–6) occurs when the specimen size is specimen. A finite element analysis of the stage shows that it reduced to the micrometer scale. Dislocations have been attrib- suppresses any misalignment error in loading by six orders of uted to cause both of these behaviors as long as the grain size or magnitude (27). The clearance (XXЈ) between the fixed and the thickness is Ͼ100 nm. At smaller length-scales, strain- moving parts allows spring-loading of the stage on a TEM gradient-dependent strengthening disappears (7) and reverse straining stage and prevents any prestress on the specimen. Hall–Petch behavior is observed (8–13). Another less under- stood size effect observed at nanoscale is the reduction of Results and Discussions Ϫ Young’s modulus, which has been attributed to specimen density All of the films tested in this paper are dc sputtered [1 ϫ 10 6 (13) and grain boundary compliance (14, 15). Models that torr (1 torr ϭ 133 Pa) base pressure, 2.7 mtorr Argon pressure, attempt to explain the nanoscale size effect are of two basic 300 W, room temperature], 99.99% pure, and deposited on bare types: (i) models describing nanocrystalline materials as two- silicon substrate. The films are subjected to compressive residual phase composites with grain interiors and boundaries, where the stress. Hence, the free-standing specimen buckles (Fig. 1) and mechanical properties are averaged by simple ‘‘rule of mixtures’’ relieves most of the stress. Because the critical buckling load is (16, 17); and (ii) models considering dislocation motion (10, 18, small, the compressive stress in the specimen after buckling is 19), grain boundary sliding (20, 21), and diffusion (12, 22) as negligible compared to the tensile stress applied during testing. competing deformation mechanisms. Although the literature The microinstrument is first used to apply tensile stress on has compelling evidence of these size-effects, the underlying 100-nm-thick free-standing aluminum films in situ in a Philips deformation mechanisms are not yet well understood (23). CM200 TEM at 160 kV. The average grain size of the films was 50 nm. The experiments were performed quasistatically, and Experimental Methods microstructures were visually monitored continually with digital Direct experimental observations of the deformation mecha- image acquisition. TEM images were recorded as single frames nisms mentioned above while the materials behavior is measured after each increment of strain of the sample. No video camera quantitatively is difficult at the nanoscale even with the recent was used for recording. Fig. 2 shows the stress–strain diagram for novel existing approaches (24–28). We overcome the difficulty by developing microinstrumentation that combines quantitative tensile testing of thin films with the qualitative capabilities of the This paper was submitted directly (Track II) to the PNAS office. transmission electron microscope (TEM) so that one can simul- Abbreviation: TEM, transmission electron microscope (microscopy). taneously measure the stress–strain states in solids and observe ‡To whom correspondence should be addressed. E-mail: [email protected]. the deformation mechanisms during materials testing. Although © 2004 by The National Academy of Sciences of the USA www.pnas.org͞cgi͞doi͞10.1073͞pnas.0400066101 PNAS ͉ April 27, 2004 ͉ vol. 101 ͉ no. 17 ͉ 6335–6340 Downloaded by guest on October 1, 2021 Fig. 1. (a) Scanning electron micrograph of the microstage showing the freestanding aluminum thin-film specimen being attached to the force sensor beam at one end and to supporting beams at the other. Markers D and F, read displacements at both ends of the specimen. The left end of the microstage is pulled by a piezo actuator of a TEM straining stage. Deflection of the sensor beam (read by marker F), multiplied by its spring constant, gives the force on the specimen. The difference between the two markers’ (D and F) gaps gives the elongation of the specimen. The gap XXЈ prevents accidental straining of the sample during mounting of the microstage on to the TEM straining stage. The TEM straining stage has to move and deform the microstage to close the gap XXЈ before loading the sample. (b) Microtensile test stage on a TEM straining stage. (c) Zoomed view of the specimen and the two displacement sensors D and F. There is no substrate under the specimen so that the TEM beam can go through the sample for microstructural inspection. three specimens tested in TEM and one in scanning electron loading direction. Elastic modulus for the four samples is found microscope to highlight the consistency of the experimental to be 68 GPa, which is close to the bulk value of 70 GPa. The films results. Also shown are TEM images of a sample at different had ͗111͘ texture. levels of stress and strain, and during failure. The top right TEM The TEM images show neither any dislocation activity within the image shows the film after failure with a crack normal to the grains nor any generation even at high stress. However, one cannot Fig. 2. Quantitative, in situ TEM test results for 100-nm-thick freestanding aluminum films. Three TEM test results are shown along with a scanning electron microscope test result to demonstrate experimental consistency. (Insets) Microstructures corresponding to the different stress–strain states for experiment 1 (triangle data markers). The rightmost Insets show crack growth through the grains and between the grains. The top right Inset shows the film after fracture. 6336 ͉ www.pnas.org͞cgi͞doi͞10.1073͞pnas.0400066101 Haque and Saif Downloaded by guest on October 1, 2021 ENGINEERING Fig. 3. (a) Low elastic modulus and nonlinear elastic response with small plastic deformation in small-grained but thick gold film. (b) As the grain size of gold is increased while the film thickness is decreased, bulk gold behavior is approached (elastic modulus of bulk gold with ͗111͘ texture is 80–87 GPa, depending on isostrain and isostress condition). (c) Low elastic modulus and nonlinear elastic response in small-grained Al. (d) Reduced permanent strain hardening in 65-nm-grain-sized aluminum (150-nm-thick film), but macroscopic strain hardening in 212-nm-grained-sized (480-nm-thick film) samples. Resolutions in stress measurements are as follows: 2.4 MPa for 350-nm-thick Au, 5 MPa for 50-nm-thick Al, 9 MPa for 150-nm-thick Al and 200-nm-thick Au, and 8 MPa for 485-nm-thick Al. Resolution in strain measurement for all of the tests is 0.05%.
Details
-
File Typepdf
-
Upload Time-
-
Content LanguagesEnglish
-
Upload UserAnonymous/Not logged-in
-
File Pages6 Page
-
File Size-