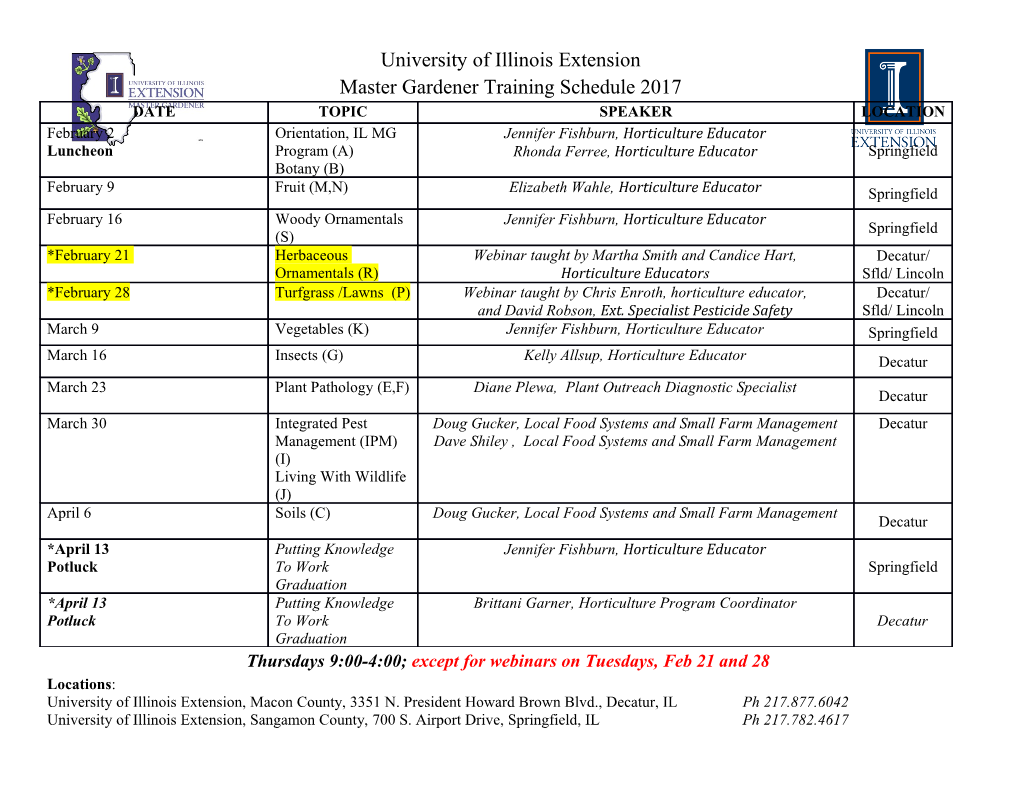
Theory and Applications for Sulfur Chemistry: Hydrogen from Hydrogen Sulfide by Ryan J. Gillis B.S., Brigham Young University (2015) Submitted to the Department of Chemical Engineering in partial fulfillment of the requirements for the degree of Doctor of Philosophy in Chemical Engineering at the MASSACHUSETTS INSTITUTE OF TECHNOLOGY September 2020 ○c Massachusetts Institute of Technology 2020. All rights reserved. Author................................................................ Department of Chemical Engineering July 30, 2020 Certified by. William H. Green Professor Thesis Supervisor Accepted by . Patrick S. Doyle Graduate Officer, Department of Chemical Engineering 2 Theory and Applications for Sulfur Chemistry: Hydrogen from Hydrogen Sulfide by Ryan J. Gillis Submitted to the Department of Chemical Engineering on July 30, 2020, in partial fulfillment of the requirements for the degree of Doctor of Philosophy in Chemical Engineering Abstract In this thesis, I explore the chemistry of reacting sulfur species computationally and experimentally. The computational work centers around creating the capability to automatically predict the thermochemical properties of arbitrary sulfur molecules and the kinetic parameters of reactions between these species. A demonstration of this enhanced capability is shown in the automatic creation of detailed chemical mech- anism describing the partial oxidation of dimethyl sulfide. The experimental work focuses on a hydrogen generating chemical cycle that uses a hydrogen sulfide feed- stock. Initially exploring the reactivity of hydrogen sulfide, water, and iodine mixtures to form hydroiodic acid, two competing pathways were discovered. The more inter- esting pathway involved the reaction of hydrogen sulfide with iodine and water to form hydroiodic acid and sulfur dioxide. A bench-top prototype was created demon- strating the creation of hydrogen gas from hydrogen sulfide through this pathway. Technoeconomic modeling of the proposed process was conducted, suggesting both commercial and environmental motivation for adoption. The thesis concludes with a brief discussion of future work. Thesis Supervisor: William H. Green Title: Professor 3 4 Acknowledgments At the end of this doctoral work, it often seems to me both that I just arrived at MIT and that I have only hazy memories of my life before coming to Cambridge. My wife is the most important person in my life. I often feel incomplete without her. Her patience as I spent long hours writing and in lab enabled any success found in these pages. My son, Diego, is both the bane of my sleeping hours and the delight of my waking ones. Although the life of an often absent father is the only one that he has known, his shy smile and eager gurgles provide me with both the drive to create and hope for a better world. My parents and siblings also deserve mention for the impact that they had through my formative years. The love of learning and sense of responsibility they instilled have led me to where I am today. Perhaps most impactful on this work is my adviser, Bill. His kindness, knowledge, and willingness to let me work on problems that I felt were important have shaped my development as a scientist, engineer, and human being. I have also been fortunate to have found a home in many different communities at MIT and in Cambridge. My time as a Graduate Resident Tutor at McCormick has enriched my life with the many wonderful students that have become dear and close friends. The Green research group has also provided a model of collaborative work and learning. Filled with individuals smarter than I, they have always been a willing resource for my many questions and thoughtful collaborators. Finally, my faith community in the local Latter-day Saint congregation has been a family to me despite the thousands of miles from my siblings and parents. 5 6 Contents 1 Introduction 25 1.1 Sulfur: An Element of Contrasts . 26 1.2 Sulfur Chemistry in Nature . 26 1.2.1 Occurrence . 26 1.2.2 Similarities and Differences . 28 1.3 Sulfur Chemistry in Industry . 30 1.3.1 Sources of Sulfur . 30 1.3.2 End Uses of Sulfur . 32 1.4 Hydrogen from Hydrogen Sulfide . 34 1.4.1 Hydrogen Sulfide’s Properties . 35 1.4.2 Motivation . 36 1.5 Review of Lab Tested Methods . 38 2 Advances in Mechanism Prediction for Sulfur Species 43 2.1 Introduction . 43 2.2 Methods . 45 2.2.1 Quantum Chemical Calculations and Dataset Creation . 45 2.2.2 Mechanism Generation . 46 2.3 Results . 47 2.3.1 Benchmarking of the Calculation Procedure . 47 2.3.2 Dataset . 49 2.3.3 Thermochemistry Prediction . 50 2.3.4 Dimethyl Sulfoxide Oxidation . 51 7 2.4 Discussion . 53 2.4.1 Discrepancies between the Model and Experiment . 53 2.4.2 Sensitivity Analysis of Major Species . 56 2.5 Conclusion . 57 3 Hydrogen from Hydrogen Sulfide and Water 59 3.1 Introduction . 59 3.1.1 Hydrogen from Hydrogen Sulfide . 59 3.2 Methods . 61 3.3 Results . 64 3.3.1 HI Formation Rate at Low H2S Concentrations . 65 3.3.2 HI Formation Rate at High H2S Concentrations . 68 3.3.3 Concentration Regimes of the Branching Pathways . 69 3.3.4 Experimental Characterization of the Sulfur Products . 70 3.3.5 Computational Evidence . 71 3.4 Discussion . 74 3.4.1 H2 production from H2S and H2O ............... 74 3.4.2 Thermochemical H2S Decomposition to Elemental Sulfur . 77 3.5 Conclusion . 78 3.6 Acknowledgment . 78 3.7 Author Contributions . 78 4 Prototype Construction and Testing 81 4.1 Introduction . 81 4.2 Safety Considerations . 81 4.3 System Inventory . 83 4.3.1 Material Selection . 85 4.3.2 Reactor Details . 86 4.4 Analytic Techniques . 88 4.5 Initial Testing Results . 89 4.6 Conclusion and Next Steps . 90 8 5 Techno-economic Modeling and Process Viability Examination 93 5.1 Introduction . 93 5.1.1 Thermochemical Cycles . 94 5.1.2 Aspen Plus Modeling . 96 5.1.3 Experimental Methods . 97 5.2 Hydrogen Productions from Hydrogen Sulfide with Elemental Sulfur Byproducts . 97 5.2.1 Water Solvent Process Model . 97 5.2.2 Solvent Substitution Experiments . 101 5.2.3 Isopropyl Alcohol Solvent Process Model . 103 5.2.4 Solvent Selection . 106 5.2.5 Process Viability and Comparison . 107 5.3 Hydrogen Productions from Hydrogen Sulfide with Sulfur Dioxide Byprod- ucts . 108 5.3.1 Flowsheet . 108 5.3.2 Energy and Utilities . 112 5.3.3 Results and Viability . 113 5.4 Conclusions . 116 6 Summary and Recommendations for Future Work 117 6.1 Summary . 117 6.2 Recommendations for Future Work . 118 6.2.1 Sulfur Chemistry Expansion . 118 6.2.2 Sour Gas Processing . 119 6.3 Final Conclusion . 120 A Tables 121 A.1 Group Additivity Values . 122 A.2 Molecule Dataset . 140 A.2.1 Validation Set Comparison . 140 A.2.2 Full Molecule List with Thermochemistry . 142 9 A.3 Kinetics . 166 A.3.1 High Pressure Rate Constant Expressions . 166 A.4 Experimental Appendix . 172 A.4.1 Experimental Conditions . 172 A.4.2 Experimental Index . 173 A.5 Computational Appendix . 174 A.5.1 Gas Phase Thermochemistries . 174 B Figures 193 B.1 Table of Contents . 193 B.1.1 Transition States . 193 B.1.2 Additional Sensitivity Plots . 215 B.2 Experimental Details . 215 B.2.1 Side reactions . 215 B.2.2 Kinetic Inference on the Iodine Concentration Dependence . 217 B.2.3 Sulfur Product Analysis . 218 B.3 Computational Details . 224 B.3.1 Thermochemistries . 224 B.3.2 Branching Points . 225 10 List of Figures 1-1 Illustration of the biogeochemical interconversion pathways for sulfur[125] 27 1-2 Analogous sulfur and oxygen-centric molecules. 29 1-3 Examples of sulfur molecules that seemingly break the octet rule. 29 1-4 Sulfur sources in the U.S. and Internationally in 2017.[11] . 33 1-5 Sulfur consumption by end us in the U.S. in 2017.[11] . 34 2-1 The molecules in the validation set. 46 2-2 A histogram sorting the deviations between experiment and calculation for 49 sulfur and oxygen containing molecules. The two outliers were excluded when determining the BAC values. 48 2-3 Parity plots comparing the calculated thermochemical value and the linear model prediction . 50 2-4 A diagram of the key reaction pathways for the oxidation of dimethyl sulfide by hydroxyl radicals. CH3S(O)OH, OCS and CH2O were observed but not quantified in the experiments[7][15]. 52 2-5 A. Comparison of the experiment and model at 280K and 1 bar of synthetic air over the course of the experiment. B. Comparison of the terminal selectivities of the experiment and model at 260K, 270K, 280K, 290K, and 298K. 53 2-6 Pathways for dimethyl sulfone formation in the model. None fully explained the dimethyl sulfone formation observed in the experiments. 54 11 2-7 Top three most sensitive kinetic and thermodynamic parameters for dimethyl sulfone formation. Thermodynamic sensitivities are reported mol in units of kcal ............................... 55 2-8 Top three most sensitive kinetic and thermodynamic parameters for thioformaldehyde formation. Thermodynamic sensitivities are reported mol in units of kcal ............................... 55 2-9 Top three most sensitive kinetic and thermodynamic parameters for dimethyl sulfoxide formation. Thermodynamic.
Details
-
File Typepdf
-
Upload Time-
-
Content LanguagesEnglish
-
Upload UserAnonymous/Not logged-in
-
File Pages244 Page
-
File Size-