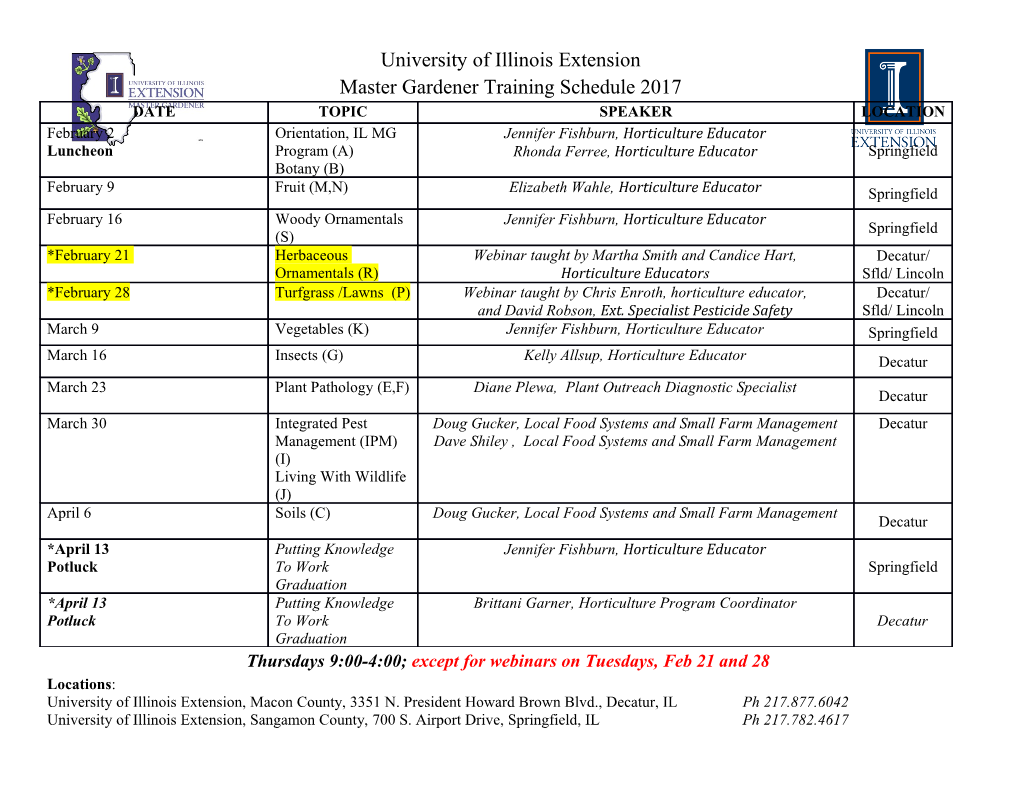
GCEP Technical Progress Report April 2013 Project: Capturing Electrical Current via Microbes to Produce Methane Alfred M. Spormann, Professor, Department of Chemical Engineering, and of Civil and Environmental Engineering, Stanford University; Bruce Logan, Professor, Department of Civil and Environmental Engineering, The Pennsylvania State University Svenja T. Lohner, postdoctoral researcher, Department of Civil and Environmental Engineering, Stanford University Michael Siegert, Douglas F. Call, postdoctoral researchers, Dept. of Civil and Environmental Engineering, The Pennsylvania State University Abstract The proposed microbial electromethanogenesis cell (MEMC) process will allow for the cost effec- tive production of methane from CO2 produced, for example, from gas/coal powered power plants or anaerobic digestion of bio-waste. The MEMC technology used will allow for production of CH4 with high efficiency relative to the electrical input. The technology is currently at the con- cept stage, with the scientific feasibility proven. However, the underlying science fundamentals such as the molecular and microbiological mechanisms of electron transfer from cathodes via in- termediates to cells and of the stability of the cathodic microbial communities are only insuffi- ciently understood. This lack of understanding presents the most significant bottleneck that needs to be solved before such technology can be deployed. The proposed research will advance both the science and the technology of this process, and the outcome should enable commerciali- zation of this technology. Background Recently, it has been discovered that CO2 can be directly converted to methane by microorganisms that harvest electrical energy from a cathode of a microbial electrolysis cell (Cheng et al. 2009). While the mechanism(s) behind “electromethanogenesis” are not fully understood, the experiment has been repeated by independent laboratories and is currently under development for industrial applications. The central issue is the potentials needed for this process. Direct electron transfer via electromethanogenesis has so far required cathode potentials more negative than approximately 0.8 V. However, indirect methanogensis can occur at much more positive potentials, resulting in less energy input. Our hypothesis for this GCEP project is therefore that in situ hydrogen production improves methane generation rates (mediated route using H2) compared to electromethanogenesis (direct transfer). Biofilm formation on the cathode can enhance methane production, and therefore one of the central questions examined here is which materials are best for methane production in these bioelectrochemical reactors. Results 1. Microbial electromethanogenesis via H2 as intermediate- efficient production of H2 To investigate abiotic hydrogen formation and biotic methane formation we built two-chamber microbial electrolysis cells (MEC; Figure 1). MEC reactors allow water-splitting reactions at the 1 Figure 1: Schematic drawing of a two- cathode to produce hydrogen which is then consumed by methanogenic microorganisms to produce methane from CO2. CO2 is dissolved in an aqueous medium as the only carbon source. Using this method, CO2 can be recycled to fuel. This method requires input of electrical energy and is therefore ideal for storing off-peak renewable energy when it is generated but not demanded. Abiotic hydrogen evolution during water splitting reactions at a cathode can well explain Figure 1. MEC experimental set-up. methanogenesis. Here, we conducted experiments to show that increased hydrogen production at cathodes in MECs indeed improves methane formation. We recorded hydrogen formation rates of 7 different catalysts. During the experiment, we observed that the catalyst with the highest hydrogen formation rate also produced methane at the highest rate ( Figure 2). Figure 2: Abiotic hydrogen production rates with different catalysts (left) and their respective methane production rates. After the reactors were allowed to generate hydrogen, the medium was replaced by a medium that contained nutrients and microorganisms able to produce methane. The methanogenic microorganisms grew in the cathode compartment using hydrogen, produced by the cathode, as their energy source and CO2 as carbon source. The catalyst with the highest hydrogen and 2 methane production rate also had the lowest electrical overpotential for hydrogen formation. Other catalysts did not produce significantly more methane than controls that were unable to evolve hydrogen. This shows that hydrogen was the intermediate in electromethanogenesis and that other mechanisms (direct electron transfer) were insignificant. In conclusion, our experiments demonstrated that the production of hydrogen along with low electrical overpotential is the most energy efficient way to produce methane in MEC reactors. 2. Microbial electromethanogenesis via defined strains To test and distinguish between H2-dependent and H2-independent microbial electromethanogenesis, different strains of methanogenic archaea were used in experiments in the bioelectrochemical reactors. 90 mL mineral medium were filled into each chamber of a 140- mL H-cell glass reactor (Fig. 1). The anode and cathode compartments were separated by a cation exchange membrane and graphite rods were used as cathode and anode materials. After sterilization of the reactor set-up, methanogenic cells pregrown on hydrogen and CO2 were harvested, washed and resuspended in the cathode chamber. The cathode was poised at -600mV vs. SHE and current consumption was monitored. In addition, hydrogen as well as methane formation was measured via gas chromatography. Fig. 3 shows the results for one of the strains tested, although they were similar for all methanogens investigated. Methane formation started without a lag-time and ,445.67"189:/76"4/96;085<""#)!!=>"?6@ABA"A98;78@7":C7@/D6;"65619@/76" methane continued to *!" increase linearly over a ,-./01"1B@@6;9" time course of more than !" two weeks. Hydrogen production (not shown) )!" #!$!%" was observed in the +' beginning 100 h und then )* #!$&" leveled off presumably due +'0%'1$234526$' to its consumption by ")./ !"##$%&'( '( (!" methanogens. At the same - #!$&%" !, time a cathodic current of about 0.1 mA was #!$'" measured throughout the ,-./01"23+" !" experiment. Comparison !" %!" &!!" &%!" '!!" '%!" (!!" (%!" +!!" to abiotic control reactors 70)$'(1+' confirmed that no abiotic Fig. 3: Current consumption and stable methane formation in methanogens with or electrochemical a graphite cathode poised at -600mV vs SHE. Red lines show current consumption and methane production for the abiotic control at -600mV vs SHE. methane formation occurred and no cathodic current above background was detected. Also we found that with more positive potentials such as -400mV vs SHE methane formation was negligible compared to -600mV vs SHE. 3 From the products gained during electromethanogenesis (hydrogen and methane), the current efficiency for this process could be evaluated as shown in Fig. 4. From the current profile electron consumption could be determined and electrons recovered in methane and hydrogen could be calculated according to following equations: + - CO2 +8H +8e → CH4 +2H2O − - 2 H2O + 2e → H2 + 2 OH B66+5,4"-=.>04,"60.,1C=+D""E%!!?F"3,/272"2.=14=/4">A4/0G,1",+,-./04," '$!" '!!" 9=.>045-",+,-./012"-0127?,4" '#!" &!" 97//,1.",@-5,1-A" '!!" &!" %!" %!" $!" $!" *+,-./012"/,-03,/,4"51"6/047-." !"##$%&'$()*$%)+',-.' 89:$;":#<" #!" #!" !/%0"1$234$)/5$#$2'67$)&#/%0',!.' !" !" !" (!" '!!" '(!" #!!" #(!" )!!" )(!" $!!" 8*1$',9.' Fig.4: Electron balance for electromethanogenesis showing consumed electrons at the cathode (green), recovered electrons in products. (purple) and current efficiency (blue). From this, a maximum current efficiency of about 40-65% could be determined varying between different strains and experimental conditions. Future experiments will focus on closing the gap in the electron balances as well as increasing the current efficiency for electromethanogenesis. Another set of experiments focused on comparing different types of graphite cathodes, in particular varying their surface area. Reactors with the same methanogen but different graphite cathodes (graphite rods, graphite brushes and graphite granules) were set up and cathodes were poised at -600 mV vs SHE. Methane formation was monitored for several days and methane production rates were derived from the linear part of the curves. As depicted in Fig. 5 the type of cathode had a significant effect on the methane production rate. As expected the graphite granules resulted in the highest methane formation due to their larger surface area compared to the brushes and rods. Within the pores of the granules there is much more surface area available for the microbes to attach or directly interact with the electrode surface. However, at the same time it was difficult to perform an electron balance with the graphite granules as after applying the cathode potential a large inrush current occurred that exponentially decayed before it leveled 4 off. The exponential decay of the initial current suggests a capacitance-like behavior, which might be attributed to surface charging effects. As this renders a current efficiency calculation impossible, we decided to work primarily with graphite rod electrodes until this problem is resolved. In summary we could demonstrate a stable '" methane formation from cathodic electrons &#(" for two weeks at -600mV vs SHE. In addition we
Details
-
File Typepdf
-
Upload Time-
-
Content LanguagesEnglish
-
Upload UserAnonymous/Not logged-in
-
File Pages5 Page
-
File Size-