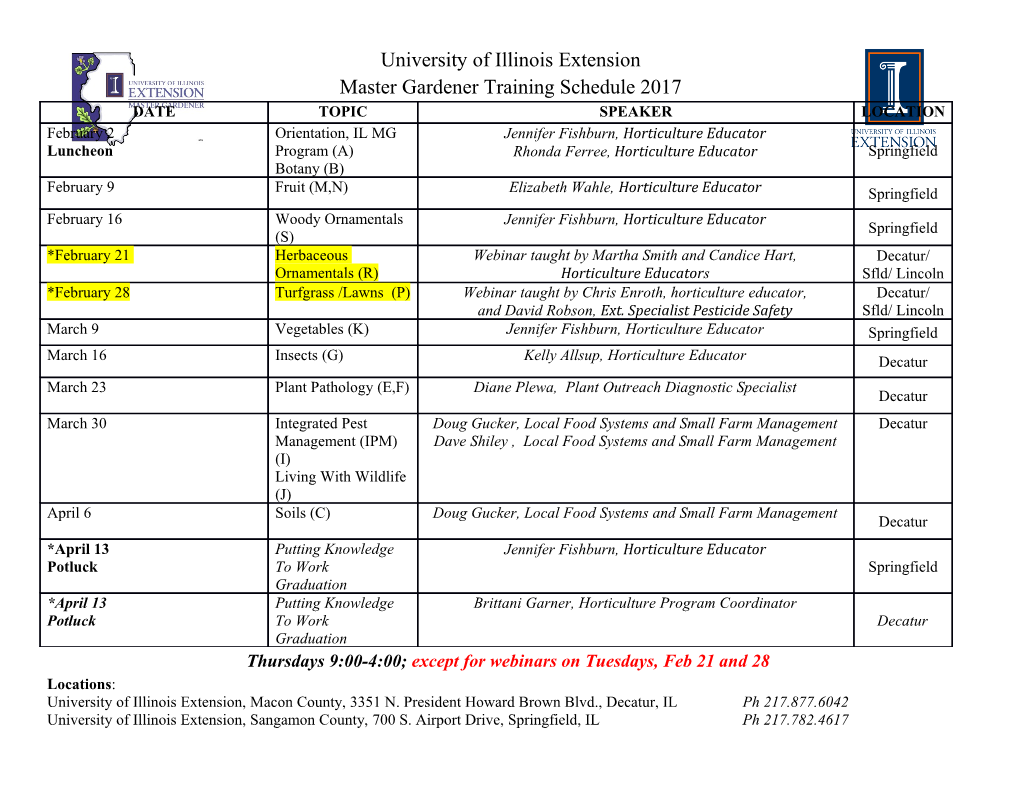
CHARACTERIZATION OF COPPER ELECTROPLATING AND ELECTROPOLISHING PROCESSES FOR SEMICONDUCTOR INTERCONNECT METALLIZATION by JULIE MARIE MENDEZ Submitted in partial fulfillment of the requirements For the degree of Doctor of Philosophy Dissertation Advisor: Dr. Uziel Landau Department of Chemical Engineering CASE WESTERN RESERVE UNIVERSITY August, 2009 CASE WESTERN RESERVE UNIVERSITY SCHOOL OF GRADUATE STUDIES We hereby approve the thesis/dissertation of _____________________________________________________ candidate for the ______________________degree *. (signed)_______________________________________________ (chair of the committee) ________________________________________________ ________________________________________________ ________________________________________________ ________________________________________________ ________________________________________________ (date) _______________________ *We also certify that written approval has been obtained for any proprietary material contained therein. TABLE OF CONTENTS Page Number List of Tables 3 List of Figures 4 Acknowledgements 9 List of Symbols 10 Abstract 13 1. Introduction 15 1.1 Semiconductor Interconnect Metallization – Process Description 15 1.2 Mechanistic Aspects of Bottom-up Fill 20 1.3 Electropolishing 22 1.4 Topics Addressed in the Dissertation 24 2. Experimental Studies of Copper Electropolishing 26 2.1 Experimental Procedure 29 2.2 Polarization Studies 30 2.3 Current Steps 34 2.3.1 Current Stepped to a Level below Limiting Current 34 2.3.2 Current Stepped to the Limiting Current Plateau 36 2.3.3 Effect of Current Density 38 2.3.4 Effect of Rotation Speed 40 2.4 Highly Resistive Surface Film 42 2.5 Electrochemical Impedance Spectroscopy 44 2.6 Stability of the Film in Presence of Chloride 49 2.7 Two-Compartment Cell Experiments 51 2.8 Conclusions 52 3. A Mechanistic Model for Copper Electropolishing 53 3.1 Regime I – Buildup of Surface Copper Ion Concentration 53 3.2 Regime II – Controlling Transport through a Surface Layer 57 3.3 Model Verification 60 3.3.1 Time Delay Prior to the Onset of the Sharp Potential Increase 67 3.3.2 Effect of Water Concentration on the Limiting Current 67 3.4 Conclusions 72 4. Novel Polyether Suppressors Enabling Copper Metallization of High Aspect Ratio Interconnects 73 4.1 Experimental Procedure 75 4.2 Results and Discussion 76 4.2.1 Polarization Data 79 4.2.2 Modeled Via-fill Ratio 89 4.2.3 Interaction with the Anti-suppressor 96 4.3 Conclusions 98 1 5. Mechanistic Studies of Polyether Adsorption 99 5.1 Experimental Details 101 5.1.1 Attenuated Total Reflectance Fourier Transform Infrared Spectroscopy (ATR-FTIR) 101 5.1.2 Quartz Crystal Microbalance (QCM) 103 5.2 ATR-FTIR Studies of PEG Adsorption 106 5.3 Quartz Crystal Microbalance 118 5.4 Effect of Cu+ on Copper Deposition 125 5.5 Conclusions 127 6. Major Conclusions and Recommendations for Future Work 128 6.1 Major Conclusions 128 6.1.1 Electropolishing 128 6.1.2 Novel Polyethers Extending Gap-fill Capabilities 129 6.2 Recommendations for Further Studies 131 Bibliography 135 2 LIST OF TABLES Page Number Table 2.1. Ohmic and polarization resistances measured by electrochemical 48 impedance spectroscopy. Table 3.1. Copper electropolishing model parameters. Justification for the 61 estimated values is given in the text. Table 4.1. Polyethers explored in the polarization studies. 77 Table 4.2. Kinetics parameters fitted to polarization data in Figure 4.1 and 90 Figure 4.2. 3 LIST OF FIGURES Page Number Figure 1.1. Schematic detailing the dual Damascene process. Both (a) the 17 via and (b) the trench are etched into the insulator. (c) A diffusion barrier layer and copper seed layer are both deposited by physical vapor deposition. (d) Copper is electrodeposited to fill the via and trench. (e) The overburden copper is removed by chemical mechanical planarization (CMP). Figure 1.2. Schematic of the transport and diffusion processes inside the 19 via. (a) PEG adsorbs quickly but is diffusion limited; therefore, it adsorbs primarily at the top of the via sidewalls. SPS diffuses quickly but adsorbs more slowly than PEG and adsorbs primarily at the via bottom. (b) As the fill progresses, the bottom surface contracts. The SPS becomes more concentrated at the bottom, bringing about rapid growth at the via bottom. Figure 2.1. Schematic of concentration profiles in the two major proposed 28 mechanisms for copper electropolishing. In mechanism (a), an acceptor species (indicated as water) diffuses towards the anode, complexes there with the discharged cupric ions, and diffuses back (as a complex) toward the bulk solution. According to mechanism (b), the concentration of cupric ions increases at the anode until the solubility limit is reached, at which point, a solid film is formed on the anode. Cupric ions then diffuse towards the bulk across a mass transport boundary layer of thickness δ. Figure 2.2. Polarization curves for electropolishing of a copper disk 32 electrode at various rotation speeds (A – 50 rpm, B – 100 rpm, C – 200 rpm, D – 400 rpm, E – 600 rpm) in 85 wt% phosphoric acid. The potential was scanned at 10 mV/s. Figure 2.3. Limiting current density for phosphoric acid solutions of various 33 water concentrations at 800 rpm. The linear relationship between limiting current density and bulk water concentration has led numerous investigators to associate it with the transport of an acceptor species (water) toward the anode. Figure 2.4. Potential response to a current pulse at 100 rpm (a) below the 35 limiting current (6.3 mA/cm2), and (b) at the limiting current (19.6 mA/cm2). The current is stepped up to the specified value at 100 s, held at that value for 400 s, and then stepped down to zero at 500 s. Note that the potential scales in (a) and (b) are quite different. 4 Figure 2.5. Potential transient responses to current steps from zero to three 39 values on the limiting current plateau. The current was stepped to (A) 18.8 mA/cm2; (B) 17.8 mA/cm2; (C) 17.0 mA/cm2 at 100 s. The disk was rotated at 100 rpm. Figure 2.6. Potential responses to currents steps from zero to 19.6 mA/cm2 41 (at the limiting current) for various rotation speeds: A – 90 rpm, B – 100 rpm, C – 110 rpm. The time delay prior to the sharp potential increase shows a strong dependence on the rotation speed. Figure 2.7. Nyquist plots at various applied potentials below the limiting 46 current at 400 rpm. The ohmic resistance remains approximately constant, but the polarization resistance decreases as the potential is increased. Figure 2.8. Nyquist plots at an applied potential of 1.3 V vs. copper (at the 47 limiting current plateau) and various rotation speeds. These measurements indicate an ohmic resistance of approximately 2.8 Ω-cm2 and a polarization resistance between 1.6 and 3.4 Ω-cm2, which decreases with increasing rotation speed. Figure 2.9. Potential response to current step of 14.2 mA/cm2 (near the 50 limiting current) in 85 wt% H3PO4 solution containing 100 ppm HCl. The potential oscillations suggest the formation and breakdown of a film. Figure 3.1 Schematics representing (a) Regime I and (b) Regime II of the 54 proposed model. In Regime I, the concentration at the anode increases until Csat is reached. In Regime II, a flux imbalance leads to the buildup in thickness (x) of a surface film. Figure 3.2. Comparison of measured and modeled overpotential response to 63 a current pulse in Regime I. The measured data are from Figure 2.4a, while the predicted response is based on the numerical solution of Eqs. [3.1] and [3.5]. The two curves are in reasonable agreement. The small deviation can probably be attributed to the spatial distribution of the copper ions. Figure 3.3. Comparison of the model governing Regime II (Eq. [3.12]) to 65 the experimental potential response displayed in Figure 2.4b (region C-D). Note the highly expanded time scale. Figure 3.4. Sensitivity analysis for parameters A and M (Eq. [3.12]). The 66 lines indicate values for these parameters such that the model correlates the data from Figure 2.4b (region C-D) within the indicated percentages. 5 Figure 3.5. The model (Eq. [3.22]) predicts a nearly linear relationship 71 between the limiting current density and the bulk water concentration for copper electrodissolution in phosphoric acid. Also indicated is a linear approximation for Eq. [3.22]. Figure 4.1. Polarization data for solutions containing 0.5 M CuSO4 (pH~2), 80 70 ppm Cl-, and 100 ppm of the specified polyether, all with molecular mass of approximately 1000 g/mol. The points for polyoxyethylene lauryl ether (diamonds) and polyoxyethylene cetyl ether (circles) fall nearly on top of one another. Figure 4.2. Polarization data for solutions containing 0.5 M CuSO4 (pH~2), 82 70 ppm Cl-, and 100 ppm of the specified polyether with molecular mass of approximately 600 g/mol. Figure 4.3. Polarization data for additional solutions containing 0.5 M 83 - CuSO4 (pH~2), 70 ppm Cl , and 100 ppm of the specified polyether with molecular mass of approximately 600 g/mol. Figure 4.4. Polarization data for solutions containing 0.5 M CuSO4 (pH~2), 85 70 ppm Cl-, and 100 ppm of the specified polyether with molecular mass of approximately 300 g/mol. Figure 4.5. Polarization data for solutions containing 0.5 M CuSO4 (pH~2), 86 70 ppm Cl-, and 100 ppm of the specified polyether with molecular mass in the range of approximately 2000 g/mol to 4000 g/mol. Figure 4.6. Overpotentials at 5 mA/cm2 as a function of the number of ether 88 oxygen atoms in the polyether. The circles correspond to PEG, and the squares are all other polyethers studied (listed in Table 4.1).
Details
-
File Typepdf
-
Upload Time-
-
Content LanguagesEnglish
-
Upload UserAnonymous/Not logged-in
-
File Pages140 Page
-
File Size-