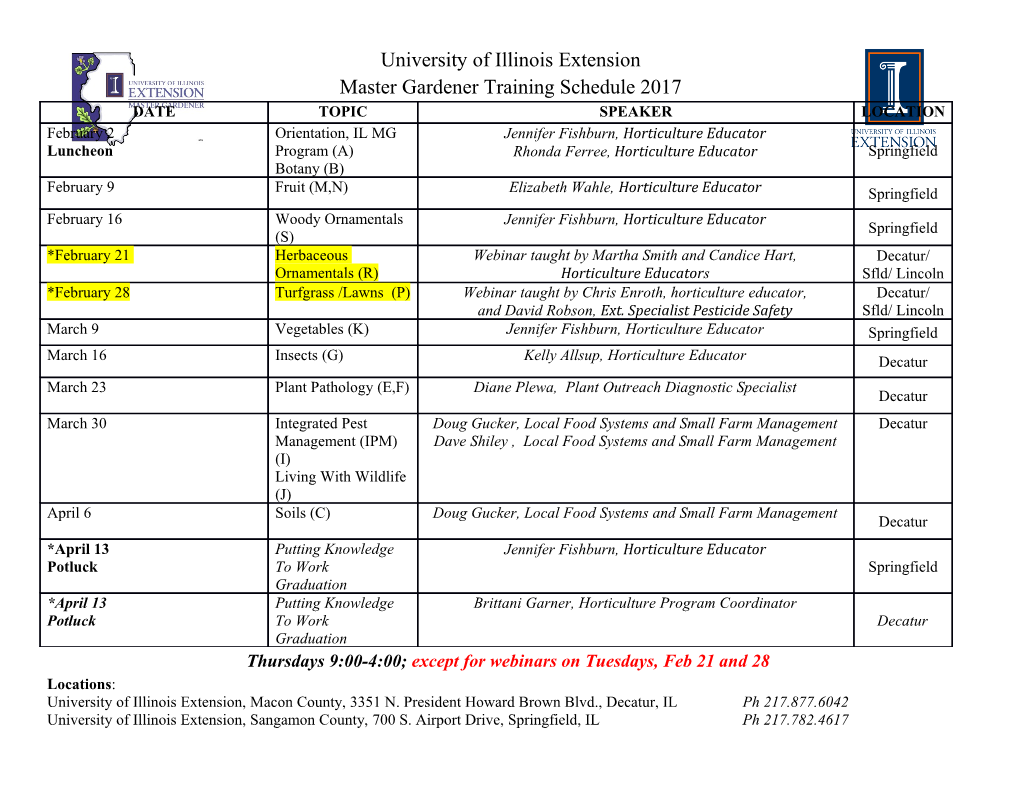
371 Reduction of Energetic Demands through Modification of Body Size and Routine Metabolic Rates in Extremophile Fish Courtney N. Passow1,* Keywords: adaptation, cave environments, energy consump- † Ryan Greenway1, tion, extreme environments, hydrogen sulfide springs, Poecilia Lenin Arias-Rodriguez2 mexicana, resource availability. Punidan D. Jeyasingh1 † Michael Tobler1, 1Department of Zoology, Oklahoma State University, 501 LifeSciencesWest,Stillwater,Oklahoma74078;2División Académica de Ciencias Biológicas, Universidad Juárez Introduction Autónoma de Tabasco, Villahermosa, Tabasco, Mexico Animals require energy for maintenance, growth, and re- Accepted 2/13/2015; Electronically Published 3/23/2015 production, and since individuals’ energy expenditure may be greater or less than the environmental energy availability, they Online enhancements: appendix figures and tables. can modulate a variety of physiological processes to balance energy supply and expenditure (Cho et al. 1982). Metabolic rate is a physiological measure of the rate at which organisms burn calories from assimilated food resources to produce en- ABSTRACT ergy for organismal functioning, and understanding meta- Variation in energy availability or maintenance costs in ex- bolic rate variation is critical for investigating ecological pro- treme environments can exert selection for efficient energy use, cesses at multiple levels of organization (Brown et al. 2004; and reductions in organismal energy demand can be achieved Sibly et al. 2012). The majority of metabolic rate variation in in two ways: reducing body mass or metabolic suppression. animals coincides with variation in body mass and tempera- Whether long-term exposure to extreme environmental con- ture (Peters 1983; Gillooly et al. 2001; Brown et al. 2004; Clarke ditions drives adaptive shifts in body mass or metabolic rates and Fraser 2004; Cano and Nicieza 2006). Nonetheless, mass- remains an open question. We studied body size variation and and temperature-adjusted metabolic rates can vary substan- variation in routine metabolic rates in locally adapted popu- tially even among closely related taxa (McNab 1986; Clarke lations of extremophile fish (Poecilia mexicana) living in toxic, and Johnston 1999; Nagy et al. 1999; Lovegrove 2000; Schaefer hydrogen sulfide–rich springs and caves. We quantified size and Walters 2010). Elucidating theselectiveforcesthatshape distributions and routine metabolic rates in wild-caught in- such residual metabolic rate variationinallometricplotsand dividuals from four habitat types. Compared with ancestral underlie macroevolutionary patterns in the diversification of populations in nonsulfidic surface habitats, extremophile pop- metabolic rates is a critical challenge in physiology (Garland ulations were characterized by significant reductions in body and Carter 1994; Feder et al. 2000). size. Despite elevated metabolic rates in cave fish, the body size Resource availability is a strong source of selection driving reduction precipitated in significantly reduced energy demands in adaptive modification of metabolic rates within and among all extremophile populations. Laboratory experiments on com- closely related species (Mueller and Diamond 2001; McCue mon garden–raised fish indicated that elevated routine met- 2010; Moiroux et al. 2012). From an energetic point of view, abolic rates in cave fish likely have a genetic basis. The results fitness can be described as the conversion rate of energy into of this study indicate that adaptation to extreme environments offspring (Brown et al. 1993), which ultimately is limited first directly impacts energy metabolism, with fish living in cave by the rate at which organisms can acquire energy from the and sulfide spring environments expending less energy overall environment and then by the rate at which they can allocate during routine metabolism. energy to reproduction (as opposed to maintenance or growth). Consequently, reductions in environmental resource availabil- ity or increases in maintenance or growth costs are predicted *Corresponding author. Present address: Division of Biology, Kansas State Uni- to constrain the amount of energy for reproduction and exert versity, 116 Ackert Hall, Manhattan, Kansas 66506; e-mail: [email protected]. selection for a reduced overall energy demand that allows for †Present address: Division of Biology, Kansas State University, 116 Ackert maximizing relative energy allocation to the production of Hall, Manhattan, Kansas 66506. offspring. Organisms can reduce their overall energy demand Physiological and Biochemical Zoology 88(4):371–383. 2015. q 2015 by The in two fundamental ways. First, they can reduce their body University of Chicago. All rights reserved. 1522-2152/2015/8804-4021$15.00. size by reducing energy allocation to growth, which simul- DOI: 10.1086/681053 taneously reduces total energy expenditure for maintenance This content downloaded from 129.130.037.136 on February 23, 2016 12:33:42 PM All use subject to University of Chicago Press Terms and Conditions (http://www.journals.uchicago.edu/t-and-c). 372 C. N. Passow, R. Greenway, L. Arias-Rodriguez, P. D. Jeyasingh, and M. Tobler (Blanckenhorn 2000; Wikelski and Romero 2003; Pafilis et al. dividuals and by sexual selection through ecotype-assortative 2009; McNab 2010). Second, organisms can reduce metabolic mating (Tobler 2009; Tobler et al. 2009b; Riesch et al. 2011a). rate independently of body size, which changes the allometric The strikingly different environmental conditions in this relationship between metabolism and body size (Guppy and system are expected to profoundly affect organismal energy Withers 1999; Wang et al. 2006; Burton et al. 2011). It is im- budgets. Caves have been widely considered as energy limited portant to note that these mechanisms are not mutually ex- because of a lack of photosynthetic primary production (Poul- clusive but may work in synchrony, such that focusing merely son and White 1969; Langecker 2000), and some cave organ- on body size or metabolic rates alone can lead to erroneous isms accordingly have evolved lower metabolic rates (e.g., conclusions (McNab 1999, 2002; Van Voorhies et al. 2004; Mc- Hüppop 1985, 1986; Hervant et al. 2000; Poulson 2001; Wil- Cue 2010). helm et al. 2006). Similarly, exposure to H2S has been shown Systems in which closely related populations occur in habi- to constrain energy acquisition in P. mexicana (Tobler et al. tats with starkly different environmental conditions provide 2009a). H2S causes and aggravates hypoxia in natural envi- an excellent opportunity to study evolutionary change in or- ronments (Bagarinao 1992), driving exposed fish to trade off ganismal energy demand. This is especially true for species time between benthic foraging and aquatic surface respira- that have invaded extreme environments, and comparisons tion, which directly mediates survival in the toxic and hypoxic between populations in localized extreme environments and environment (Plath et al. 2007b; Tobler et al. 2009a). In ad- adjacent “benign” habitats allow for a powerful approach to dition, exposure to perpetually sulfidic environments requires examine the effects of stressors on organismal physiology as active detoxification for survival. Sulfide spring fish exhibit a fi well as the evolutionary trajectories of populations. Exposure heritable and constitutive increase in H2Sdetoxi cation abil- to physicochemical stressors profoundly affects energy bud- ity through upregulation of the sulfide:quinone oxidoreduc- gets of organisms because the maintenance of homeostasis tase pathway (Tobler et al. 2014), a process that is energetically precipitates in considerable energetic costs through invest- costly (Ip et al. 2004; Hildebrandt and Grieshaber 2008). Ac- ments in physiological, morphological, or behavioral coping cordingly, some organisms adapted to sulfidic environments mechanisms (Calow 1989; Sibly and Calow 1989; Parsons have been documented to increase energy consumption in the 1996). As such, continuous exposure to environmental stress- presence of H2S (Gorodezky and Childress 1994; Schneider ors should select for increased metabolic rates (e.g., Kno- 1996). Clearly, exposure to extreme environmental conditions blauch et al. 1999). However, the evolution of increased meta- impacts organismal energy budgets through reduced energy bolic rates in extreme environments is typically constrained by availability, reduced ability for energy acquisition, and/or in- a reduced supply of resources required for metabolic expen- creased organismal maintenance costs, which is reflected in diture (Waterman 1999, 2001). While many studies have in- P. mexicana from both sulfidic and cave environments con- vestigated immediate metabolic costs of exposure to physi- sistently having a lower body condition than fish from non- cochemical stressors and energy limitation (e.g., Haney and sulfidic surface habitats (assessed through abdominal dis- Nordlie 1997; Penttinen and Kukkonen 1998; Rose et al. 2006; tension [Plath et al. 2005], body fat content [Tobler 2008], and Wang et al. 2006; McKenzie et al. 2007; McCue 2010), it re- mass-length relationships [Tobler et al. 2006]). Consequently, mains unclear how metabolic rates and metabolic rate plas- adaptation to these environments should be linked to energy ticity evolve when populations adapt to diverse environmental metabolism, and we hypothesized that extremophile popu- stressors. Here, we explicitly tested for differences in body size lations should be selected for reductions
Details
-
File Typepdf
-
Upload Time-
-
Content LanguagesEnglish
-
Upload UserAnonymous/Not logged-in
-
File Pages13 Page
-
File Size-